Introduction to Gene Therapy and Related Techniques for Retinal Disorders and Age-Related Macular Degeneration
Henry Alexander Leder
Keisuke Mori
Peter L. Gehlbach
INTRODUCTION
Gene therapy is the introduction of exogenous nucleic acids into a host’s cells with subsequent expression for the treatment of disease. Gene therapy can be thought of as a pharmacologic method by which nucleic acids and ultimately bioactive proteins become the therapeutic agents delivered to the target tissues. Among the therapeutic benefits arising from gene transfer are gene repair, gene activation or inactivation, and the expression of proteins that are therapeutic to the disease state. Gene expression cassettes are designed for the production of therapeutic proteins, noncoding RNAs, or the constituents of other novel treatment strategies. Many of these strategies are currently in the basic or preclinical stages of development. Gene expression cassettes can be transferred into target cells by a variety of methods, one of the most frequent being viral transfection. Once the expression cassette is introduced into the target tissue, the foreign DNA is expressed by the host cell. Bioactive products that result may be therapeutic to the host cell or in the case of secreted proteins may be therapeutic to adjacent cells or distant diseased tissues.
Gene therapy had received much of its early theoretical support by the early 1970s (before the recombinant DNA era) from knowledge of the mechanisms of cell transformation by tumor viruses. Classes of DNA and RNA tumor viruses have evolved that carry out precisely those functions crucial to gene therapy, that is, the heritable and stable introduction of functional new genetic information into mammalian cells. Thus, it was proposed that such viruses or other like agents, deprived of their own deleterious functions, could be used as vehicles to introduce normal and functional genes into human cells to correct cellular defects and cure genetic diseases (1).
The first human gene therapy trial was begun in September 1990 and involved transfer of the adenosine deaminase (ADA) gene into lymphocytes of a patient having an otherwise lethal defect in this enzyme; the
disease produces immune deficiency. The results of this initial trial were encouraging and have helped to stimulate further clinical trials (2).
disease produces immune deficiency. The results of this initial trial were encouraging and have helped to stimulate further clinical trials (2).
It is essential to know the precise structure, function, and regulation of a target gene for successful gene therapy. General steps for gene therapy include the following: First, identifying the defective gene and isolating a normal counterpart. Second, the normal gene must be inserted into a cell where it must function properly. This may require integration into the host cell chromosome or deletion of the defective gene. An isolated, cloned gene can either be directly injected into a cell or be packaged into a vector by recombinant DNA techniques and then introduced. Once in the cell, it may become integrated into the nuclear DNA or remain free in the cytoplasm as a self-replicating, extrachromosomal element (3). Finally, the transferred DNA must be expressed by the host cells, resulting in a bioactive product. Some gene therapy techniques require cell mitosis for efficient incorporation of the therapeutic gene into the host chromosome. Retroviral vectors (e.g., lentivirus, a modified HIV vector) have a demonstrated ability to transfect postmitotic adult retinal neurons.
A number of ocular diseases are amenable to gene therapy approaches, and the eye has characteristics that are potentially advantageous to these strategies. In brief, the eye is a small compartment and is relatively isolated by virtue of its ocular coats and a blood-ocular barrier. Therefore, intraocular injection of a small amount of vector results in transduction of a relatively large proportion of susceptible ocular cells and relatively little entry into cells outside of the eye. This makes it possible to minimize systemic side effects.
An important application of gene transfer techniques is in the replacement of mutated genes that code for proteins needed for the normal functioning of ocular cells. In the case of defective photoreceptors or retinal pigment epithelial cells, gene transfer provides a way to replace the defective gene product and potentially treat various retinal degenerations (4,5,6). This type of gene therapy holds great promise for the treatment of inherited disorders causing blindness such as retinitis pigmentosa and Leber congenital amaurosis (LCA) and may someday play a role in treating the genetic aspects of age-related macular degeneration (AMD) (7).
An alternative gene transfer strategy is to transduce cells within the eye in order produce therapeutic proteins that do not replace specific defective proteins. Rather, the overexpressed protein may serve as a survival factor that rescues or protects, for example, photoreceptor cells from a variety of neurodegenerative processes that might result in retinal degeneration. Alternatively, the expression of an antiangiogenic factor might be expected to broadly inhibit a number of diseases via inhibition of angiogenesis as a final common pathway for a number of ocular diseases. Pigment epithelium-derived factor (PEDF) is an example of a molecule that has been shown to be of protective value in preclinical evaluation using a number of animal models of angiogenesis and neurodegeneration, following ocular transduction with viral vectors (8,9,10,11,12). Both adenovirusvectored PEDF and a lentivirus-vectored somatostatin have been in phase I clinical evaluation.
THE RETINA AS A GENE TRANSFER TARGET
Retinogenesis is a developmental process that is tightly regulated both temporally and spatially and is therefore an excellent model for studying the molecular and cellular mechanisms of neurogenesis in the central nervous system (13).
The retina has unique properties that make it particularly amenable for gene transfer. It is a thin laminar structure that is accessible by multiple routes; consequently, different areas of the eye and various cell types are accessible to therapeutic intervention. Fortuitously, the fact that the eye is small and enclosed limits the dose of vector necessary to achieve a local therapeutic effect. There are physical barriers to the retina, such as the blood-retinal barrier, that further protect from widespread dissemination of the vector into the systemic circulation. The subretinal space is separated from its choroidal blood supply by the retinal pigment epithelium (RPE) and its intercellular junctions and is protected from the interior of the eye by the internal limiting membrane and the retina itself. These barriers provide a beneficial effect in protecting the retina from the immune response by sequestering antigens from the systemic circulation but also can make access to subretinal space more challenging. The eye as a treatment target possesses experimental advantages for viral vector delivery; for example, due to the size of the globe, one can reasonably extrapolate a treatment dose from an animal experiment to a human study. This extrapolation is more reliable than for other organ systems. In addition, there are a number of sophisticated means to evaluate retinal therapeutic efficacy over time. Retinal function can be monitored with noninvasive and quantitative tests that include but are not limited to ophthalmoscopy, electroretinography, optical coherence tomography, the measurement of afferent pupillary responses, and visual evoked potentials. Finally, a number of genes responsible for retinal disease have been identified, and a number of animal models of retinal disease exist and continue to improve and new models based on increasing understanding of disease continue to evolve (14).
RETINAL DEGENERATION
Retinal diseases amenable to gene therapy are often characterized by neuronal degeneration such as retinitis pigmentosa or Stargardt’s disease or by complications of disease
such as ocular neovascularization in AMD and other retinal diseases. A significant advance has been the discovery of the ABCR (retina-specific, ATP-binding cassette transporter) gene and its mutation in autosomal recessive Stargardt’s macular dystrophy and fundus flavimaculatus (15). The ABCR gene is expressed in rods; its gene product, rim protein, is thought to function in the recycling of rod outer segment components between the RPE and the retina (1). Carriers of heterozygous gene defects may be at increased risk for AMD; several ABCR gene mutations have been identified in some populations of patients with AMD. In addition, mutations in TIMP3 lead to Sorsby’s fundus dystrophy, a retinal disease that exhibits characteristics of AMD, including thickened Bruch’s membrane, high risk for neovascularization, and photoreceptor degeneration (16).
such as ocular neovascularization in AMD and other retinal diseases. A significant advance has been the discovery of the ABCR (retina-specific, ATP-binding cassette transporter) gene and its mutation in autosomal recessive Stargardt’s macular dystrophy and fundus flavimaculatus (15). The ABCR gene is expressed in rods; its gene product, rim protein, is thought to function in the recycling of rod outer segment components between the RPE and the retina (1). Carriers of heterozygous gene defects may be at increased risk for AMD; several ABCR gene mutations have been identified in some populations of patients with AMD. In addition, mutations in TIMP3 lead to Sorsby’s fundus dystrophy, a retinal disease that exhibits characteristics of AMD, including thickened Bruch’s membrane, high risk for neovascularization, and photoreceptor degeneration (16).
DOMINANT AND RECESSIVE RETINAL DEGENERATIONS
Retinal diseases may have multifactorial causes. Inherited retinal degenerations may be sporadic, dominant, recessive, X-linked, or maternal (mitochondrial). In addition, they differ in onset, function of the gene product, pattern and level of gene expression, region of the retina affected, primary and secondary cells involved, and clinical manifestations. Ideally, it would be beneficial to develop a therapy that would permanently correct the defect at the genomic level. To date, researchers have largely focused on a gene replacement approach for recessive mutations and a gene inactivation approach for dominant mutations (14).
Recessively inherited retinal degenerations represent a group of retinal abnormalities whose phenotype is due to the lack of function of a gene; in other words, they are characterized by the inability to produce a functional gene product. The absence of the normal gene product, such as a structural protein of the outer segment or an enzyme in the phototransduction cascade, results in expression of the mutant phenotype. The principle of gene therapy in recessive degeneration is to “cure” the disease by replacing the missing gene with a wild-type copy in each photoreceptor. The goal of photoreceptor rescue requires efficient transduction of most of the photoreceptor cells because, in theory, any cell expressing a wild-type gene will survive. Ali et al. (17) reported the first successful rescue in rds null mice. These animals fail to form outer segments, develop an early loss of retinal function, and their degeneration is characterized by progressive photoreceptor cell death. Following adeno-associated vector delivery of peripherin/RDS, functional and morphologic studies revealed that there was outer segment restoration and proper disc formation. Somatic therapies aimed at enhancing survival using growth factors, and antiapoptosis genes have also been shown to improve photoreceptor cell survival in recessive degenerations but are not curative.
On the other hand, autosomal dominant retinal degenerations are characterized by the production of abnormal protein in photoreceptor cells. The abnormal, mutant form of the protein is, in some way, toxic to the retina and causes progressive retinal degeneration, with the final common pathway being apoptotic cell death. For this reason, gene therapy for dominant disease is focused on inhibiting the translation of the mutant protein, for example, by direct cleavage of the mutant messenger RNA (mRNA) transcript.
Several animal models of dominant retinal diseases have been generated. These transgenic animals carry one copy of a mutated gene that encodes a misfolded or mislocalized protein. The expression of these proteins in photoreceptors results in toxicity, which leads to cell death through an apoptotic mechanism. Therefore, it is strategic to eliminate or correct the mutated gene in order to restore function. The former has been successfully achieved with ribozymes in the P23H transgenic rat. Ribozymes are RNA-cleaving RNA molecules that specifically remove defective mRNAs or replace them with the correct sequence. The ribozymes were designed to target the mutant P23H transcript and be placed into an adenoassociated vector, under the regulation of a rhodopsin promoter. The treatment resulted in morphologic and functional rescue (14).
In general, retinal degenerations are variable entities varying from early to late onset and with rapid to slowly progressive courses. Slowly progressive variants may have a much wider therapeutic window than those with an earlier onset, such as Leber’s congenital amaurosis. In the former case, it is critical to use a delivery vehicle that allows for expression over extended periods of time, understanding that an eventual readministration could be necessary. It remains to be understood how best to optimize these treatments once begun. Consequently, it will be necessary to fully determine the mechanisms by which these molecules exert their protective function as well as the best routes of administration (e.g., subretinal or intravitreal) and the therapeutic/toxic dose ratio and therefore a great deal of work is ongoing.
TYPES OF POTENTIAL HUMAN GENETIC INTERVENTION
For the sake of discussion of human genetic intervention, four general categories of procedures have been outlined in accordance with their goal and target cells (18).
Somatic gene therapy: As applied to the treatment or prevention of disease, this type of intervention involves the correction of genetic defects in any of the body’s cells, with the exception of the germ or reproductive cells. Given the recent developments in the field of somatic gene therapy, it is appropriate to broaden the definition to include genes that can be introduced into cells to provide
a new function. One example of such an approach involves the insertion of a cytokine gene, such as interleukin-2 or tumor necrosis factor, into a patient’s malignant cells to produce an immune response against the tumor. Depending on the location and orientation of the recombination targets, the recombination result could be the integration, excision, inversion, or translocation of the nuclear DNA (13).
a new function. One example of such an approach involves the insertion of a cytokine gene, such as interleukin-2 or tumor necrosis factor, into a patient’s malignant cells to produce an immune response against the tumor. Depending on the location and orientation of the recombination targets, the recombination result could be the integration, excision, inversion, or translocation of the nuclear DNA (13).
Germinal gene therapy: This genetic intervention involves the correction or prevention of genetic deficiencies through the transfer of properly functioning genes into reproductive cells. To achieve the desired results from this approach, it may be necessary to replace or suppress the faulty gene rather than simply add a new gene. In germ-line alteration, gene addition would be unsatisfactory because it is not yet possible to predict the effects of a mixture of the normal gene and the mutated gene with respect to regulatory signals necessary for normal growth and development. Germ-line genetic modification could be effected either before fertilization or in the early postfertilization stages of embryogenic development.
Somatic cell or germ-line gene modifications may also be considered in order to affect selected physical and mental characteristics, with the aim of influencing such features as physical appearance or physical abilities. A principal difference in these uses of genetic modification is that they could be directed toward healthy people who have no evidence of genetic deficiency disease. Further, germ-line genetic intervention, if successful, could allow the enhancement to be passed on to succeeding generations.
STRATEGIES IN GENE THERAPY
Three general approaches are currently utilized:
1. Ex vivo: A procedure in which the new gene is transferred to cells in the laboratory and the modified cells are then administered to the recipient. This technique overcomes the problem of intraocular cell targeting. This cell therapy approach has several potential advantages. First, it improves the efficiency of transduction, selection, and cloning of transduced cells, which are more easily accomplished in vitro. Second, this approach permits assessment of safety profiles of modified cells prior to medical application. The main disadvantages of ex vivo transduction is that certain cells, such as photoreceptors, cannot yet be removed and surgically reintroduced into the retina.
2. In vivo: This gene transfer generally relies on a vector to introduce a therapeutic gene into the target cell. The vector is generally delivered remote from the target cell; for instance, the vector is delivered intravitreously, and the target cells may be the photoreceptors in the retina.
3. In situ: The gene or the vectors are delivered in the vicinity of the target cells.
In these strategies, the transfer process is usually aided by a vector that helps deliver the gene to the intracellular site where it can function appropriately (19).
The choice of an ex vivo, in vivo, or in situ strategy and of the vector used to carry the gene is dictated by the clinical target.
Gene Replacement, Correction, or Augmentation
One form of gene therapy would involve specific removal of the mutant gene sequence and its replacement with normal, functional genomic material. An ideal approach, gene correction, would entail specific correction of a mutant gene sequence without any additional changes in the target genome (1). An alternative strategy is gene augmentation, which is modifying the content or expression of mutant genes in defective cells by introducing a foreign normal genetic sequence. During the past decade, a number of efficient methods have been developed to introduce functional new genes into mammalian cells. In many cases, it is possible to restore a genetic function by the addition of nontargeted but functional genetic information into nonspecific sites of the genome without the removal or correction of a resident, nonfunctional mutant gene. During the creation of animal models for human genetic disease, inactivation of the target gene, socalled gene knockout, has been shown to be a successful methodology.
GENE THERAPY TECHNIQUES
There are a number of advantages to utilizing a gene transfer approach to therapy. One advantage is that the protein of interest may be continuously expressed over an extended duration, following a single injection of vector. The duration of protein expression varies depending on a number of factors including the type of vector used. In general, however, the duration of increased protein expression greatly exceeds the period of elevated protein levels that are achieved by a single injection of purified protein.
Currently, a number of viral vector platforms are in development and are being evaluated for potential use in ocular gene therapy. Of these, adenovirus, adenoassociated virus (AAV), retrovirus, and lentivirus are being intensively investigated by a number of laboratories around the world. Each vector is characterized by a unique biology as well as a unique set of advantages and disadvantages that relate to their potential utility as gene therapy vectors. A full discussion of each vector is beyond the scope of this chapter, but it is evident that with greater understanding and continued technologic innovation, each continues to evolve as a potential therapeutic strategy in the eye.
VIRAL VECTORS
Adenovirus Vectors
Adenoviruses are nonenveloped, double-stranded DNA viruses that infect a broad range of human and nonhuman cell types by binding to specific cell surface receptors. By a process of endocytosis, the virus gains access inside the target cell, then the virus binds to the nuclear envelope, and enters the nuclear space. The virus is not incorporated into the host genome but remains as a transcriptionally active episome within the nucleus. Concerns about the infectious nature of adenoviral vectors have led to the development of replication-deficient (helper-dependent) adenoviruses for gene transfer. Deletion of the replication-specific genes and nonessential DNA sequences from these viral vectors prevents viral replication. Adenoviral vectors can infect both dividing and nondividing cells, which makes them particularly useful to transfer genes to postmitotic cells. Adenoviruses have been effective in transducing retinal photoreceptors, ganglion cells, Müller cells, and RPE cells but do have some inherent limitations with respect to potential clinical application in the eye. For instance, peak transgene expression is typically limited to a few weeks as there is no integration into the host DNA. The broad range of target cells is a potential disadvantage if only one cell layer or cell type is targeted. Adenoviral transduction can also induce inflammatory responses and a significant immune response to viral proteins in the host. This may in turn limit the duration of gene expression and also expose transduced cells to immunologic responses. Modified adenoviral vectors may improve the duration of gene expression by reducing the number of viral proteins presented to the host immune system (20).
Several advantageous properties of adenovirus vectors have increased their potential utility as gene therapy vectors in recent years. Pioneering “proof of principle” work in retinal gene transfer has been conducted with adenoviral vectors (4,5). Among the advantages of this vector platform are the relative ease of production and purification, resulting in purification of high titers in large quantities; their ability to accept a large cloning capacity; an ability to transduce a variety of noncycling cells; the potential for high expression levels and comparatively rapid transgene expression. The use of adenoviral vector is, however, potentially complicated by significant inflammation as well as a shorter duration of expression than other available vectors (21,22,23,24,25). Inflammation and immune responses induced by adenovirus vectors may result in destruction of transduced cells. Vector modifications to reduce the immunogenicity of this vector, such as deletion of the E1A, E1B, E3, E4 virus genes, or the use of helper-dependent adenoviral vectors that are deleted in all viral protein-coding sequences (26,27,28,29,30) results in less cytotoxicity and potentially longer duration of transgene expression.
Despite some limitations, the adenoviral DNA vectors have been shown to be effective. They are easy to apply experimentally in vitro and in vivo, and they may be particularly useful for gene therapy in which transgene expression of limited duration is sufficient to achieve the desired clinical effect (31). In addition, clinical grade second-generation Ad vectors have been shown to be well tolerated in human eyes (32).
Adeno-Associated Virus Vectors
The potential for gene delivery to the eye using AAV vectors has received much attention. AAV is a member of the Parvovirinae subfamily of Parvoviridae. It is nonenveloped, with icosahedral symmetry and a diameter of 18 to 26 nm. It was originally isolated as a contaminant of adenoviral cultures and thus given the name AAV. This human nonpathogenic virus is replication defective in the absence of a helper virus. AAV is packaged as a 4.7 kb single-stranded DNA molecule. In the absence of a helper virus or helper function, the AAV genome integrates in the host cell genome entering the latent phase of its cycle (14).
Different AAV serotypes have different virion shell proteins and, as a consequence, vary in their ability to bind to and transfect different host cell types. To date, eight different AAV serotypes have been isolated, and possibly many more may be harbored in the genome of different mammalian tissues. The most divergent AAV sequence belongs to serotype 5, which was isolated from a condylomatous lesion. AAV 7 and 8 sequences were isolated from rhesus monkey tissues. All others to date have been isolated as contaminants of adenoviral cultures (14).
AAVs have a number of important advantages over other vectors that make them suitable for ocular studies; in particular, a relative lack of pathogenicity and their ability to induce long-term transgene expression in the eye. AAV vectors can be used to transfect a variety of ocular cell types including photoreceptors, RPE cells, Müller cells, retinal ganglion cells, trabecular meshwork cells, and corneal endothelial cells.
AAV vectors and lentivirus vectors each induce little immune response and mediate long-term expression (33,34). AAV vectors are promising because they are not considered pathogenic in humans and because transduction of a wide variety of postmitotic cells, including muscle cells and certain neuronal cells, is possible. The duration of AAV-mediated expression of proteins in the human eye is not yet fully defined and varies depending on a variety of factors some of which are known and some of which are still not well understood. In rodents, there is evidence that in some settings, expression can occur for the entire life of the animal. Although such extended periods of expression are theoretically advantageous in the setting of chronic disease, the effects of excessive and prolonged expression of various transgenes in retinal cells remains largely unknown. By way of example, it is known that increased expression
of wild-type rhodopsin or peripherin/rds can result in degeneration of photoreceptors (35,36). A brief profile of the advantages of AAV as a vector platform for ocular gene therapy reveals significant advantages including highly efficient transduction of some retinal cells, stable transduction of a variety of cell types, including neurons, and the potential for prolonged duration of expression. Some of the potential benefits and risks of each of the highlighted vector platforms are shown in Table 24.1.
of wild-type rhodopsin or peripherin/rds can result in degeneration of photoreceptors (35,36). A brief profile of the advantages of AAV as a vector platform for ocular gene therapy reveals significant advantages including highly efficient transduction of some retinal cells, stable transduction of a variety of cell types, including neurons, and the potential for prolonged duration of expression. Some of the potential benefits and risks of each of the highlighted vector platforms are shown in Table 24.1.
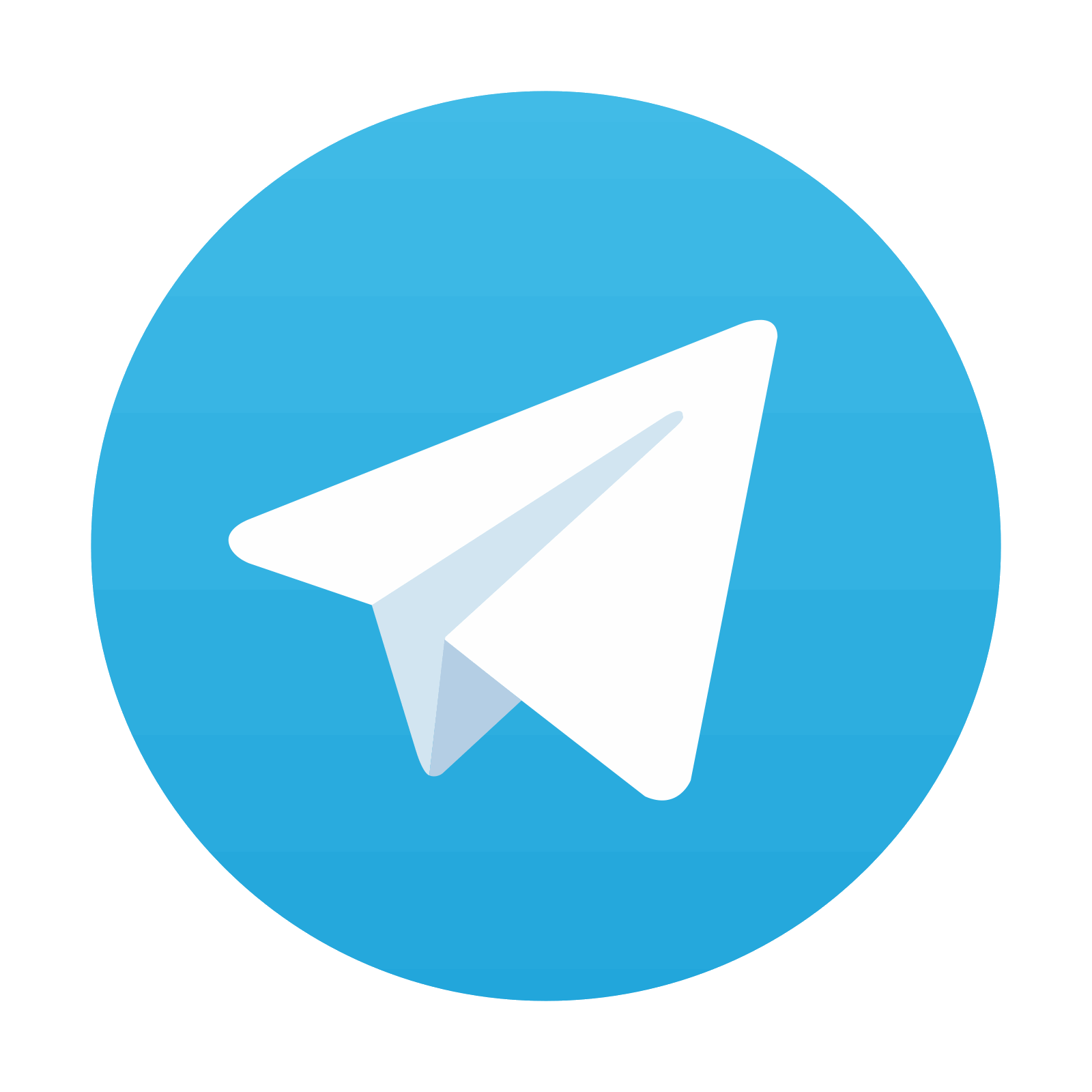
Stay updated, free articles. Join our Telegram channel
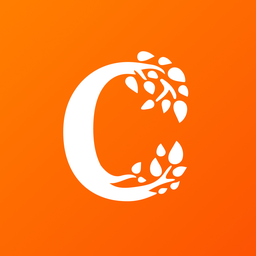
Full access? Get Clinical Tree
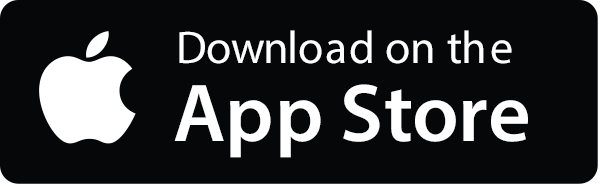
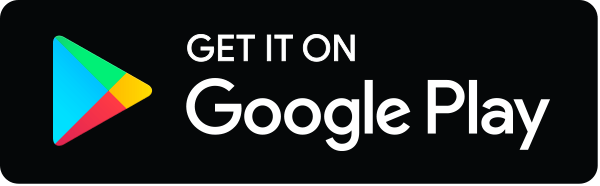