Introduction
This chapter covers the basic principles of clinical pharmacology, with particular reference to drugs used in the management of ophthalmic disorders, methods of ocular drug delivery, and the interactions of drugs and the eye. Although it is generally thought that the medical management of ocular disease is mainly administered through topical therapeutic agents, many systemic drugs and agents are also used. These include diuretics for the control of intraocular pressure, immunosuppressants for control of intraocular inflammatory conditions and antimicrobials for control of infection. Therefore, basic pharmacological principles (pharmacokinetics and pharmacodynamics) are important to ophthalmologists. The basic pharmacology of systemic therapy, including receptor–drug interactions, is reviewed before discussing the more specific topical and systemic therapies in the treatment of ophthalmic disorders.
Pharmacokinetics: drug trafficking in the body
Basic concepts
Pharmacokinetics is the mathematical study of the time-course of drug absorption, distribution, metabolism and excretion. One of the simplest parameters to consider when discussing the pharmacokinetics of any drug is the biological half-life of a drug ( ), which is the time taken for the plasma drug concentration to fall by half after administration. A more accurate method of assessing the efficiency of drug elimination is the estimation of drug clearance from the circulation. For example, after intravenous administration, the
of the drug may be calculated from its plasma concentration–time curve. This simplistic model is based on a single compartment model that states that, following intravenous administration, the distribution of a drug assumes a uniform concentration throughout all compartments (intracellular and extracellular); the elimination by both metabolism and renal excretion is also assumed to be directly proportional to the drug concentration. If this is the case, the volume of distribution may be calculated as:
V d = dose / C 0
The apparent volume of distribution is defined as the volume of fluid required to contain the total amount of drug in the body at the same concentration as that present in plasma. Drugs in general may be confined to the plasma compartment because they are either too large to cross the capillary wall or are highly protein-bound (e.g. heparin and warfarin, respectively). Drugs may also be distributed only to the extracellular compartment because they have low lipid solubility (e.g. gentamicin) or distributed throughout all aqueous compartments if they are lipid-soluble. When both the volume of distribution and renal clearance of a drug are known, the may be estimated. However, when considering a single compartment model, no account is taken of distribution into tissue compartments and metabolism within tissue compartments. When repeated injections of the drug are given, the plasma concentration becomes a function of the rate of both elimination and administration, and the plasma concentration will equilibrate when these two parameters are equal. A steady-state plasma concentration is therefore reached, which in practice may be established after an interval of about three or four plasma half-life lives of the drug given. To reach a steady-state concentration more quickly, loading doses of the drug are often given (for example, antibiotics and warfarin) ( Fig. 6-1 ). In clinical practice the
is important because it will determine the frequency of administration of a drug. If continued intravenous boluses are given, there are frequently large peaks and troughs in drug concentration, which can lead to a greater incidence of toxic side-effects.

Bioavailability describes the amount of oral dose that reaches the systemic circulation and becomes available to the site of drug action. However, this blanket term is not sufficiently precise because rapidly absorbed drugs will reach a much higher plasma concentration than those absorbed slowly and, similarly, rapid elimination would also theoretically lead to low bioavailability. Bioavailability of a drug is measured as the area under the curve of log plasma concentration against time for both intravenous and oral administration, although the reliability of quantitative drug assessment may be variable for several reasons. For instance, the degree of bioavailability may be altered by incomplete absorption of the drug or destruction of the drug by first-pass metabolism before the drug reaches the plasma compartment, irrespective of the rate of absorption from the gastrointestinal tract. Drugs instilled into the eye are absorbed from the nasal and nasopharyngeal mucosae directly into the systemic circulation. As such, they escape first-pass metabolism and have a high bioavailability. Thus, topically administered agents can give rise to quite marked systemic effects (see below).
Drug kinetics can be described as first-order (linear) or zero-order (non-linear, saturation) kinetics ( Fig. 6-2 ). First-order kinetics describes a process where rate is proportional to the amount of drug present and can be defined by linear differential equations. Zero-order kinetics occurs when drug dynamics show saturation at high drug concentrations. Saturation may occur, for example, when the capacity of drug-metabolizing liver enzymes is surpassed, leading to unmetabolized drug in the circulation for longer periods. The duration of action of a drug that exhibits saturation kinetics is more dependent on the administering dose than in drugs that exhibit first-order kinetics. Also, there is no direct relationship between drug dose and steady-state plasma concentration in zero-order kinetics, which may explain sudden unexpected drug toxicity in a number of clinical settings. For this reason, close drug monitoring is required (e.g. phenytoin).

Drug absorption
For a drug to reach the site at which it produces its effect, it must first be absorbed from its site of administration. In general, drug penetration of cell membranes increases with lipid solubility. Drugs can cross cell membranes by diffusing directly through lipid, by diffusion through aqueous pores that traverse the lipid, by utilizing carrier molecules, or by pinocytosis by the cell.
The rate of passage (diffusion) through a cell membrane can be predicted by Fick’s law
Non-polar substances dissolve freely in lipid and therefore penetrate the cell by diffusion. The lipid solubility, degree of ionization and molecular size of the drug will determine its diffusion coefficient. The rate is determined by Fick’s law, which states that the rate at which drugs cross a biological membrane is directly proportional to the concentration gradient across the membrane and the diffusion coefficient, and inversely proportional to the cell membrane thickness (see below):
Rate of diffusion = K A ( x 1 − x 2 ) / D
Active transport
As for active transport of ions (see Ch. 4 , p. 161 ), active transport of large polar drugs requires energy-dependent carrier-mediated mechanisms. These transport systems may be disrupted by inhibiting enzyme-dependent carriers or by blocking the carrier mechanism with structurally similar drugs (analogues). Facilitated transport is carrier-mediated transport that does not require energy because it does not proceed against a concentration gradient, for example glucose transport into erythrocytes.
Drug absorption is dependent on a drug’s lipid solubility
Drug properties
The absorption of a drug depends on its lipid solubility and inversely on its polarity or degree of ionization. An important factor in the degree of penetration of a drug through membranes is that many drugs are weak acids or weak bases. The more the drug is in its un-ionized form, the more likely it is to be lipid-soluble and transferred by passive diffusion through the membrane. For a weak acid or base the p K a value will determine the degree of ionization, as described by the Henderson–Hasselbalch equation.
For a weak acid the ionizing reaction is:
pH = p K a + log { [ A − ] / [ HA ] }
pH = p K a + log { [ B ] / [ BH + ] }
The molecular shape, as well as the charge distribution, of the drug molecule determines which membrane pores it may traverse. Small polar molecules, such as urea, readily traverse small aqueous pores in the membrane, thus accounting for the high permeability of cell membranes to these substances. Most drugs, however, are too large to pass through these pores.
The rate of drug absorption varies with the route of administration
The main routes of administration (besides intravenous) are oral, sublingual, rectal, topical (e.g. skin, conjunctival fornix), subcutaneous and intramuscular.
Absorption of orally administered drugs is affected by gastric pH, rate of emptying of gastric contents, presence of food, and surface area of absorptive mucosa (in disorders such as Crohn’s disease the absorptive surface area may be reduced). It is important to consider drug interactions during multiple drug therapy and their effect on drug absorption. For example, in migraine, gastric emptying is delayed, reducing the absorption of analgesics such as aspirin and paracetamol. This may be overcome with the adjunctive use of parenteral metoclopramide, which increases the rate of gastric emptying. The presence of food is generally unimportant, except in the case of tetracyclines (used to treat certain forms of external eye disease commonly associated with acne rosacea), which form insoluble salts with magnesium and calcium. Some drugs will be inactivated within the gut lumen (e.g. benzylpenicillin and insulin). Most malabsorption syndromes do not affect drug absorption, but in other disorders, such as congestive cardiac failure, drug absorption may be impaired because of the secondary gastrointestinal mucosal oedema. Factors affecting bioavailability are shown in Box 6-1 .
- •
Gut motility
- •
Intestinal pH, mucus, bile salts
- •
Enterohepatic circulation
- •
Exercise
- •
Reduced absorptive area
- •
Reduced intestinal blood flow
- •
Intestinal microflora that may metabolize some drugs
Drug distribution
Once a drug is absorbed, it has the potential to penetrate most compartments of the body so the distribution of the drug depends largely on the route of administration. Intravenous and intramuscular administration of a drug result in high drug availability. Buccal (sublingual) absorption of a drug is used to reduce the extent of first-pass metabolism by the liver (as with topically applied eye drops), which invariably occurs with orally administered drugs that reach the liver via the portal circulation. The distribution of the drug and its ability to penetrate cells is also dependent on its physicochemical properties and thus the extent of binding to tissue proteins or cell membrane receptors ( Box 6-2 ).
- •
Physicochemical properties of drug
- •
Binding to plasma proteins
- •
Binding to tissue proteins
- •
Relative blood flow to different tissues
Once the drug has reached the systemic circulation, it may become bound to circulating proteins, commonly albumin or α 1 -acid glycoproteins (for basic drugs). Protein-bound drugs are restricted in their distribution into tissues, which reduces the availability of the free drug for pharmacological effect. This, in turn, depends on the affinity of the protein for the drug. High levels of protein binding may occur with acidic drugs that are bound to albumin. If a drug is less than 90% bound to plasma proteins, changes in the plasma protein concentration make little difference to the overall amount of unbound drug in the circulation. In cases of drugs with a high binding affinity for protein, a decrease in the steady-state total concentration of the drug, and as a consequence a comparative increase in the clearance of the increased amount of free drug, would occur if for any reason protein binding was impaired. For instance, impaired plasma protein binding in the case of phenytoin results in peaks of unbound active drug in the plasma.
Basic drugs are bound in varying degrees to α 1 -acid glycoprotein, a protein that increases in concentration in certain pathological conditions such as acute inflammation. Under such conditions, the binding of basic drugs (e.g. propranolol) may be increased, thus reducing their effect.
Systemic factors that alter protein binding include hypoalbuminaemia (plasma concentration of albumin less than 25 g/L), renal failure, competition by other highly protein-bound drugs, and changes occurring during the last trimester of pregnancy, such as the diluting effect of the increased plasma volume. In general, competition for binding by other drugs is the major factor affecting distribution because adequate compensatory mechanisms can be initiated to counteract the other causes. The distribution of a drug, as mentioned above, may also be regulated by the binding of a drug to tissue proteins, a process regulated by the abundance of binding sites, affinity constants and the binding of a drug to its receptor. This in turn may give rise to a desired or undesired effect, although receptor numbers are unlikely to be high enough to alter the distribution of the drug appreciably.
In the eye, both the blood–retinal barrier and the blood–aqueous barrier (see Ch. 1 , p. 30 ) limit the distribution of drugs. Tight junctions between the retinal pigment epithelium (RPE) and endothelium of the retinal vessel endothelium give rise to a relatively impermeable barrier to water solutes and larger molecules. Under normal conditions only lipid-soluble drugs will move between the blood and retina. Similarly, the apical membranes of the non-pigmented ciliary body epithelium and the capillary endothelium of the iris are bound by tight junctions, raising a barrier to all but lipid-soluble drugs (see Ch. 1 , p. 28 ).
Drugs are metabolized to facilitate clearance
The metabolism of most drugs occurs almost entirely in the liver, enzymatically altering the drug to increase its water solubility in preparation for excretion, and simultaneously making the compound metabolically and pharmacologically active or inactive. Metabolism can affect the drug in various ways. The first is activation of the parent drug, which may itself be inactive (known as a prodrug ). The drug may be metabolized to form active metabolites, as for example in the case of diamorphine and diazepam. However, metabolism of a drug can also produce toxic metabolites that may persist in the circulation for longer than the parent molecule and thus restrict the continued use of such drugs (e.g. lidocaine). In general, most drug metabolism modifies the drug so that it can then be excreted, usually in the urine but sometimes in bile.
Drug metabolism takes place in two stages: phase I (oxidation) and phase II (conjugation). Phase I reactions are carried out by a heterogeneous group of microsomal enzymes called cytochrome P 450 , of which various forms exist ( Box 6-3 ).
This enzyme system exists in abundance in the liver but is also found in some peripheral organs, including the eye. It is important to note that the activity of these enzymes can be induced by other drugs (e.g. phenytoin and carbamazepine), which accounts for many well-recognized drug interactions. Conjugation reactions appear not to be affected by enzyme-inducing drugs to the same extent as oxidative metabolism. There are also other oxidative enzymes that are not part of the cytochrome P 450 system but which are involved in drug metabolism; these include xanthine oxidase (e.g. purine metabolism), alcohol dehydrogenase and monoamine oxidase (e.g. catecholamine metabolism). Many drugs also affect the function of microsomal enzyme systems ( Box 6-4 ).
Enzyme Inducers
- •
barbiturates
- •
phenytoin
- •
phenothiazines
- •
rifampicin
- •
griseofulvin
- •
nicotine
Enzyme Inhibitors
- •
isoniazid
- •
chloramphenicol
- •
metronidazole
- •
warfarin
- •
carbon monoxide
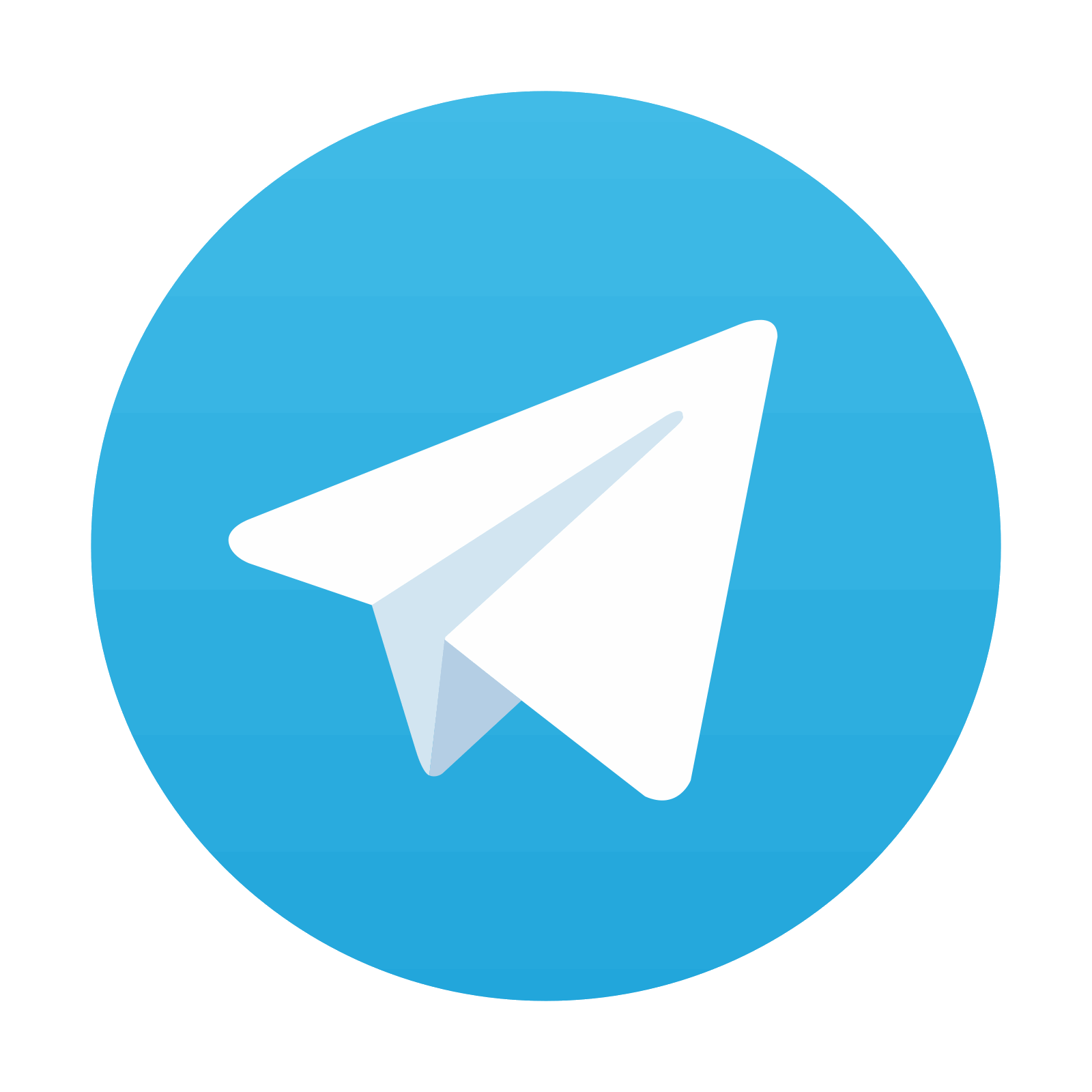
Stay updated, free articles. Join our Telegram channel
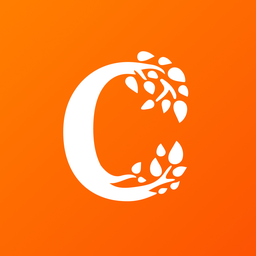
Full access? Get Clinical Tree
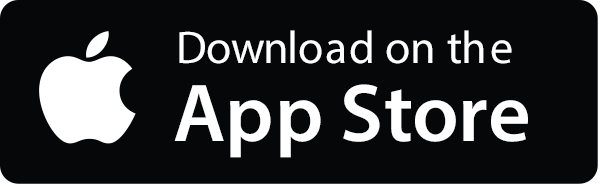
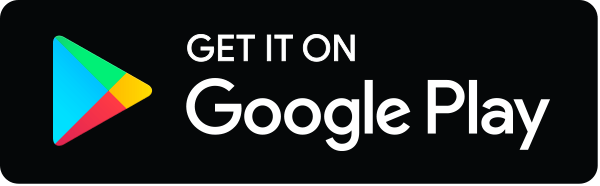
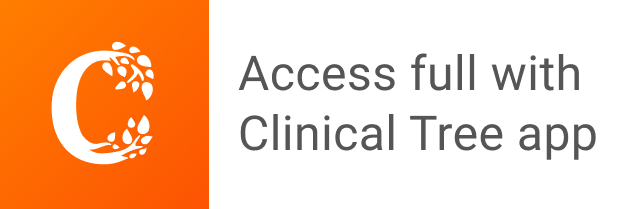