Introduction
The eye is a miracle of self-organization. While many tissues such as muscle and kidney comprise predominantly a restricted set of more or less specialized cell types, almost all cell types are represented in the eye including secretory cells, neuronal cells, vascular cells, specialized fibroblasts, tissue myeloid cells, and supporting cells, and the matrices contain all of the molecular components found in other tissues. Uniquely, the cells and tissues have been customized for the eye.
Aristotle understood the phenomenon of self-organization as it applies to many organic and inorganic systems as ‘in the case of all things … in which the totality is not, as it were a mere heap, but the whole is something besides the parts, there is a cause’ ( ). That the process of self-organization as it applies to the eye results in an organ specialized for the transmission, reception and conversion of light energy into cellular signals remains a remarkable feat of differentiation and development. Even more remarkable is the observation that the forces leading to this event appear to be intrinsic to the cells, since optic cup and eye morphogenesis can be induced in embryonic stem cells in vitro given the right conditions ( Fig. 4-1 ).

The unique feature of cells and tissues in the eye is that they are organized for the transmission, reception and conversion of light energy into cellular signals. Cells respond to stimuli in a remarkably similar manner. What differentiates one cell from another are the stimuli each cell responds to and the mechanisms it uses to respond. Cells have the genetic potential to express any type of receptor but their unique specialization is down to the limited set of membrane receptors they express. Through these receptors, cells respond to a specific stimulus by activating an intracellular second messenger system that has a limited generic range, i.e. the same set of signalling molecules is frequently activated by a wide range of ligand–receptor interactions: the specificity lies in the ligand binding. This produces a programmed response in the cell, resulting in an effect, e.g. aqueous secretion from acinar lacrimal gland cells after stimulation by adrenaline (epinephrine), an ocular muscle action potential after neurotransmitter release from nerve endings, or rhodopsin activation by a photon of light. Remarkably, the process of signal induction and transmission is based on a very limited set of biochemical reactions often involving an ATP-energy-driven mechanism, such as the addition or subtraction of a phosphate group to the signalling molecule, respectively via enzyme activity provided by a kinase or a phosphatase.
Cells are organized, singly or in groups (tissues), to receive information from the environment (via membrane receptors), to signal this information to the intracellular compartment (via signalling networks), to convert the message into cellular responses (gene activation and protein transcription) and to relay this information to the outside world (e.g. changes in cell behaviour, tissue function, secretion, etc.). Innumerable molecules and genes are involved in a single response by the cell through these networks, in which thousands of molecular interactions are connected through molecular ‘hubs’ (similar to hubs regulating aircraft traffic) (see next section). Cells may also respond simultaneously to several stimuli through several receptors, and controlling the flow of this information can be difficult. In recent years, this function seems to be attributed to that mysterious cellular constituent chromatin, whose histone tails may act as a signal storage and converter device, similar to digital–analog converter devices in the electronic industry. In this way accumulated repeated rapid ON–OFF signals over time can induce epigenetic and indeed phenotypic change in cells and tissues, which not only influence cellular functions as they age but also can be transmitted to the next generation.
Studies in mice have shown that epigenetic changes to the gene pool of the fetus can lead to damage to either the somatic cells (genes not transmitted) or the germ-line cells (genes passed on). If the damage is not sufficient to kill the fetus the first-generation progeny (F1) will only pass on their mutated genes to the next generation (F2) if the germ-line genes have been affected ( eFig. 4-1 ). If, however, there is epigenetic germ-line damage and it is stably expressed in the adult, it may be passed on as part of the normal germ-line genes (i.e. is passed down several generations – transgenerational) unless reprogramming occurs in the embryo before implantation. Reprogramming can be viewed as a type of fail-safe repair mechanism.

These concepts have been derived from the vast amount of information made available through full genetic analysis of various organisms (genomics) and the use of novel methods of investigation including microarray technology and informatics.
The continuing advance of ‘omics’
The development of systems biology, which is based on microarray techniques and high-throughput technology, has rendered the complexity of molecular interactions, such as those involved in signalling or in transcriptional regulation, amenable to analysis. This is the science of ‘omics’, a term applied to a body of work, knowledge or data, and includes genomics, transcriptomics, metabolomics, proteomics, signalomics and the microbiome. This has generated a vast amount of information leading to some further ‘omic’ subdivisions such as cancer genomics or toxicogenomics. Genomics examines the many genes which may be involved by increased or decreased expression, while transcriptomics studies the many transcription factors which may be activated or deactivated in any one cellular behaviour, such as cell division, and metabolomics investigates the many biochemical pathways which may be utilized, or not, in conversion of one molecular species to another in the process of energy generation and consumption. For the epigenetics researcher, there is even an epigenome, which provides information on DNA methylation, histone modifications and chromatin remodelling. Meanwhile, the microbiome is a term applied to the databank relating to gut commensals and has considerable influence on the immune system (see Ch. 7 , p. 373 ). In several of these ‘-omes’, molecular networks are entrained, which in themselves reveal the extensive interdependence that one system has on another. In addition, the notion of central (‘hub’-based) molecular species without which the entire network would collapse allows a hierarchy of importance to be applied to molecules. This is demonstrated in the genetic mutagenesis studies in which certain molecules, such as transforming growth factor-β, are lethal to the embryo when deleted, while others, such as plasminogen activator inhibitor 1, barely alter the murine phenotype.
Signalling networks are a prime example of how cellular information is transmitted. It is now recognized that there are hundreds to thousands of signalling receptors in the cell membrane interacting with around 10 second messenger ‘hubs’ in large interacting intracellular networks of several thousand cellular proteins (see eFig. 4-2 and eBox 4-1 ).
Many of the thousands of molecules and genes are involved in a single response by a cell through intracellular networks; in this process immense numbers of molecular interactions are connected through a system in which some molecules form many interactions with others in ‘hubs’ while other molecules make very few interactions and are on the periphery of the network ( eFig. 4-2 ). These concepts are derived from the vast amount of information made available through full genomic analysis of various organisms and the use of novel methods of investigation including microarray technology and informatics. Furthermore, hub molecules are frequently transient in activity, one molecule acting as a hub during activation of one signalling pathway while the same molecule acts merely as a relay station during activity of another pathway. Information usually proceeds from ‘outside in’ to the cell, but on occasion information initiated outside the cell can be relayed back to extracellular targets ( eFig. 4-2 ). Many of the cellular proteins may not be directly involved in signalling but may act as adaptors or amplify/diminish the overall response. In addition, other proteins act as ‘chaperones’ to protect proteins and signalling molecules for optimal function ( eBox 4-1 ).


Chaperone proteins are everywhere in the cell, shepherding essential functional proteins such as enzymes and signalling molecules to ensure their proper functioning. Many different types of chaperone exist and some come into their own in certain circumstances, such as heat-shock proteins (Hsp), while others are constitutively functioning, such as lens crystallins ( Fig. 4-2 ). Chaperones play an essential role in the activation of protein kinases: for instance, Bag1, the co-chaperone of Hsp70, which can activate the Hsp90-dependent process. Stress is known to inhibit cell proliferation and sequestration of Bag1 may be how this occurs. Chaperones such as Bag1 and Hsp70 play an essential role in the maturation and activation of hundreds of protein kinases, regulating, for instance, cell proliferation in response to stress. Chaperones participate in raft-dependent signalling of molecules such as eNOS, G-proteins and STATs. Chaperones also help the subnuclear trafficking and disassembly of transcriptional factors and related complexes.
Examples of typical general second messenger systems include:
- •
receptor tyrosine kinase-linked receptor systems (RTK)
- •
ion channels and pumps
- •
G protein-driven messengers
- •
seven-transmembrane loops.
Each of these may interact with other intracellular signalling systems and the signalling systems themselves may be ‘customized’ to respond selectively depending on the conditions ( Fig. 4-2 ). In addition, each receptor may be represented many times in a single cell (it is estimated that there are between 5000 and 10 000 major histocompatibility (MHC) class II molecules on a dendritic cell), while several different ligand–receptor pairs may act in clusters at the cell surface, as for instance in the T-cell receptor synapse (see Ch. 7 , p. 427 ).

Signalling networks behave similarly to other biological networks, such as metabolic and gene transcriptional networks, and probably represent a basic biological organizing system. Three basic concepts underpin a signalling network: signalling pathways, signalling modules and signalling nodes ( eFig. 4-2 ).
In addition, receptors exist not only on the cell surface but intracellularly on endosomes and in the nucleus and ligands may have to be transported intracellularly to interact with their receptor. Information transmitted via ligand/receptor interactions can also be bidirectional, as for instance in the regulation of stem cells in stem cell niches through integrin molecule binding to extracellular matrix proteins ( Fig. 4-3 ).

Cells and tissues
The cell
General structure
Technology drives science and there is no better example of this than the discovery of the cell as the basic unit of living organisms by Anton van Leeuwenhoek using a compound optical microscope. The basic structure of the mammalian cell can be illustrated by the retinal pigmented epithelial (RPE) cell ( Fig. 4-4 ) as it contains most of the recognized cellular structures and intracellular organelles. While all cells have the potential and machinery for mitosis and motility, many adult tissue cells such as the RPE cell are considered terminally differentiated, non-motile cells, except under pathological conditions. The RPE cell is an example of a bidirectional transporting epithelial cell with polarity, i.e. an apical surface with microvillous processes and a basal surface with numerous infoldings. The RPE is also an example of how the basic structure of the cell has been modified extensively, as in several types of specialized cells in the eye. Dysfunction of this critically important cell underlies the pathology of age-related macular degeneration (AMD), one of the commonest causes of blindness in developed nations (see Ch. 9 , p. 513 ).

The plasma membrane
The plasma membrane, which surrounds all cells, is a selective two-way barrier to passive diffusion, which also has active transport mechanisms subserved by specialized proteins (for instance ion channels, pumps and suspended transporters) floating in a lipid bilayer, composed of phosphoglycerides, sphingolipids and sterols, which forms spontaneously due to its content of phospholipids. The barrier function of the plasma membrane applies even to ions and is the basis of the electrical potential that occurs across cell membranes and which is energy-driven, for instance by which is energy-driven, for instance by ATPase enzymes. Other proteins are also suspended in the plasma membrane, such as receptors for hormones, neurotransmitters, viruses and other cells. Many of these receptors have a three-part structure with an extracellular, variably sized, component, a transmembrane component, and a short intracellular section coupled to the second messenger system. The plasma membrane is a variably thick lipid bilayer ‘crowded’ with many membrane proteins whose functions not only serve transmembrane traffic via receptors and transporters but also have a direct role to play in the physiology and indeed the survival of the cell: this is in part determined by the types of motion and tension which different membrane proteins exert ( Box 4-1 ).
Free diffusion ( A ) of proteins in the lipid bilayer allows random or directional movement depending on external triggers. Many proteins are, however, anchored or tethered either to cytoskeletal proteins or to extracellular matrix proteins ( B ). Examples include GPI-tethered proteins. Some proteins are only transiently tethered during a particular function, for instance in the immunological synapse of immune cell activation ( C ). Some of the proteins can escape from the synapse or corral and become internalized. In contrast some proteins have limited movement and are confined to a region of the plasma membrane, as in confined diffusion ( D ).

Many of these proteins are held in patchy distributions within the lipid bilayer, termed lipid (micro)domains, which themselves have variable constitution. The lipid domains also move within the membrane like rafts, and indeed if large enough are known as lipid rafts. Other microdomains decorate the cell surface in the shape of irregularly pitted invaginations and evaginations such as specialized structures for endocytosis (clathrin-coated pits) and caveolae, embedded in a glycoprotein-rich matrix (glycocalyx). These are also involved in a plethora of functions including cell signalling, protein trafficking, cell movement, waste disposal (exocytosis) and even cell survival ( Fig. 4-5 ). Microdomains are frequently detergent-resistant and usually contain a specific protein such as caveolin in caveolae, or several proteins, as occur in lipid rafts, areas specialized for specific functions such as the immunological synapse in antigen-presenting cells (see Ch. 7 , p. 421 ). Other microdomains include tiny domains (nanodomains), which contain GTP-binding protein (inhibitory) (GPI)-anchored proteins (important in some types of signalling) and glycosphingolipids, transient confined zones of varying size, and small regions composed of more fluid lipids.

Cells of the nervous system are especially rich in lipid rafts, which contain a high content of cholesterol, and sphingolipid rafts appear to have an organizational function either as discrete functional elements in which both the ligand and receptor are present, or where the activation of the receptor is dependent on recruitment to the raft of the effector ligand. It is likely that the enormous complexity associated with microdomain function will only be revealed using a systems biology approach such as a combination of proteomics and transcriptomics.
Many other specializations occur in the plasma membrane, depending on the cell type, such as junctional complexes, gap junctions, desmosomes, hemidesmosomes and contact sites with the basement membranes (see below). In the eye these membrane specializations are developed to a high level. For instance, the photoreceptor cell ( Fig. 4-4 ) (see Ch. 1 , p. 41 ) is a highly polarized structure comprising a receptor component, a nucleus and a synapse. The rod photoreceptor (specialized for scotopic vision; see Ch. 9 ) develops as an evagination of the plasma membrane, which folds upon itself many times to form stacks of membranous disks by fusion of the peripheral disk membrane. The plasma membrane is typical of any cell, i.e. it comprises a lipid bilayer containing a high concentration of membrane proteins. The lipid bilayer is a self-assembling sheet of phospholipid that adopts the bilayer format because of the physicochemical properties of the polar phospholipids, ensuring that the polar groups are external and the hydrophobic groups form the inner layer of the leaflet. The photoreceptor can adopt this special arrangement because it has more cholesterol in its bilayer, not only making it less fluid but also preventing crystallization of the membrane by inhibiting possible phase transition of the hydrocarbons. The synaptic terminal of the photoreceptor interacts with mobile Ca 2+ channels in the bipolar cell which appear to function within lipid rafts confined in their mobility by attachments to the cytoskeleton (see Part B of the figure in Box 4-1 ).
Endoplasmic reticulum and Golgi apparatus
A wide variety of cell organelles are embedded in a cytoplasmic gel, which is traversed by a system of membranes, the endoplasmic reticulum (ER). The ER, a series of thin bilayered membranes, is a flowing dynamic system constantly forming and reforming. Cisternal, tubular and vesicular elements exist. The rough ER (RER) is distinguished from the smooth ER as a ribosome-studded structure that is highly developed in secretory cells such as the lacrimal gland acinar cell, and is specialized in other cells, e.g. the sarcoplasmic reticulum of striated (including extraocular) muscle. The RER is arranged en face in rows or rosettes of ribosomes (polysomes). Newly synthesized proteins come off the ribosomes and are threaded through the lipid bilayer into the interior of the ER where they are post-translationally folded, ready for secretion via the Golgi apparatus by vacuolar budding and fusion with the plasma membrane for exocytosis ( Box 4-2 ). The smooth ER is also the site of synthesis of molecules such as lipids, triglycerides and steroids, and is prominent in cells such as the RPE and meibomian gland cells; the Golgi apparatus is a membranous stack of flattened cisternae which receives proteins, now equipped with a leader sequence required for secretion, for sorting and exocytosis from the smooth ER; the smooth ER also plays a role in lipid trafficking and modification.
Endocytosis is generally achieved via incorporation of ligand–receptor complexes in clathrin-coated vesicles. This applies to soluble proteins and to small and large particles such as viruses, which frequently use constitutive cell surface receptors to enter cells.
Clathrin-coated vesicles start as small pits on the cell surface. When the vesicle is fully intracellular it loses its clathrin coat and becomes an endosome, which fuses with primary lysosomes that have a high content of acid hydrolases and other proteases. These lead to degradation of the ingested material, and further processing depending on the cell type. Certain cell surface receptors are recycled to the cell membrane during this process to engage further extracellular ligand ( A ). Clathrin-coated pits are normally restricted to the region of the plasma membrane by the cortical cytoplasm actin organization. Relaxation of this actin assembly by proteins such as latrunculin B allows movement of the coated pits.
Exocytosis occurs by a similar process but in reverse. Cytoplasmic granules such as those in exocrine glands or in granulocytes are lipid vesicles containing material for extrusion. Secretory vesicles bud from the Golgi apparatus and are transported towards the plasma membrane by the cytoskeleton. There, they fuse in a lipid microdomain assisted by proteins such as SNARE and the SNARE-binding proteins in a ‘targeting patch’ ( B ). Fused secretory vesicle membranes act as targets for further secretory granule fusion in cells such as mast cells. In addition, vesicles termed exosomes may be ‘pinched’ off cells such as macrophages and immune dendritic cells in the same way as platelets are pinched off megakaryocytes. Exosomes convey material (information) from one cell to another and the nature of the information varies from cell to cell.

The ER and the Golgi apparatus have other functions: they are involved in signalling, for instance during mitogen activation of cells for proliferation, via the small GTPases which are activated at the plasma membrane and also signal in the ER and Golgi body ( Box 4-3 ). In fact, small GTPases and other molecules, such as phosphoinositides, provide a signature for each organelle and are involved in the specific lipid membrane folding that characterizes each organelle ( Box 4-3 ). The ER also provides a regulatory role in ensuring quality control of good protein secretion (GPS). This is regulated by a process of ubiquitinylation by which the level of ubiquitin molecules added to the protein determines whether the protein is targeted for secretion or degradation in the proteasome (see eFig. 4-3 ).
Ligand binding to the tyrosine kinase receptor (PTKR) activates the small GTPases, Ras, through a complex of signalling molecules (Grb2/SOS). Activated Ras on endosomes from the plasma membrane induces signalling in this organelle as well as in the Golgi apparatus (indirectly via phospholipase CgCa and another protein known as GRPI) while inhibition of the small Ras occurs in the endoplasmic reticulum via an inhibitor protein ERII (see figure). In this example of a signalling network, interstin and kinase suppressor of Ras serve as scaffold proteins while p14 acts as an adaptor protein in Ras-independent activation of the kinase, MEK-1 (mitogen-activated protein (extracellular signal-regulated (ER kinase) kinase), by the endosome. The integration of organelles and signalling networks with cytosolic proteins is thus central to proper functioning of the cell in response to an external stimulus such as a mitogen.

The way a protein is folded critically determines its function. This process is monitored by a ubiquitous cellular protein called ubiquitin (Ub) which is bound to the protein as it emerges from the endoplasmic reticulum. As more and more Ub molecules are added to the protein, it protects it from degradation, while as more Ub molecules are removed by the protein, it becomes susceptible to degradation and recycling/removal. This process is tightly regulated (see eFig. 4-3 ).

If proteins are not folded correctly they are unable to function and the ER generates a ‘stress response’, also known as the unfolded protein response (UPR), which is one form of ER stress. Together with a second fail-safe mechanism, mediated by a kinase known as the mammalian target of rapamycin (mTOR), the UPR and the mTOR pathways control many cellular processes including programmed cell death (apoptosis), protein translation, waste disposal (autophagy), energy supply (ATP), and response to inflammatory stimuli.
Cells respond to unfavourable conditions by demonstrating a stress response. For instance this occurs where there is an excess of free radical generation by the cell but can also occur when normal physiological mechanisms are tested, as in a condition known as ER stress. Stress is dealt with by the cell through a variety of mechanisms, including the ER stress responses and a cell signalling system known as the mammalian target of rapamycin (mTOR) response, which is implicated in many conditions involving inflammatory responses in the eye ( eFig. 4-4 ).

The ER also forms the nuclear envelope during telophase, when a series of flat vesicles surround the chromosomes and fuse at their edges. The envelope contains many nuclear pores , which are composed of eight cylindrical filamentous structures in a highly organized arrangement. Pores act as molecular sieves, permitting rapid passage of small 4.5 nm (4.5 kDa) particles and slower passage of larger molecules (12–70 kDa). The outer aspect of the nuclear envelope in secretory cells is lined with ribosomes and polysomes, while the inner surface is in contact with a nuclear filamentous matrix.
Mitochondria
Mitochondria are small (2 μm long) oval-shaped organelles comprising a two-membrane system of compartments, the inner one of which is composed of two domains, the inner boundary membrane (IBM) and the invaginations, folded into structures termed cristae ( Fig. 4-6 ). At the cristae junction with the IBM, the mitochondrion inner membrane organizing system (MINOS) is located and separates the two populations of proteins: those in the IBM assist in protein movement (translocases) and those in the cristae contain the proteins involved in the respiratory chain, the F1FO-ATP synthase and carrier proteins for ADP/ATP (see below).

The intermembrane space contains carrier proteins that are responsible for the transport of metabolites between the two compartments and also between the cytosol and the outer compartment. Their transport systems include antiport, aspartate/glutamate, ornithine/citrulline, maleate/citrate, symport, pyruvate/H + , and urea and porphyrin synthesis. Mitochondria are the powerhouses of the cell and have several essential metabolic functions as they contain all the elements for the respiratory assembly, for the citric acid cycle and for fatty acid metabolism. Their main functions therefore are to act as the site of energy-rich adenosine triphosphate (ATP)/guanosine triphosphate (GTP) formation, to function as a calcium store mainly in the form of calcium phosphate, to engage in the uptake of energy-rich substances, and to facilitate the oxidative breakdown of ATP. Mitochondria and the ER form an integrated system known as the ER-mitochondria organizing network (ERMIONE), enabling the transport of lipids and calcium between the compartments.
Mitochondria are powerhouses of nutrient handling and energy storage, essential for cell growth and proliferation. Uncontrolled, this can lead to tumours, and so a balance between homeostatic metabolism and cell death is essential. Mitochondria are therefore regulators of cell death through several routes ( Box 4-4 ).
Cytochrome c (red spots in Figure 4-7 ) in mitochondria controls many apoptosis pathways involving caspases, granzymes and perforin molecules as well as several pro- and anti-apoptotic genes such as Bax, Bcl2, BH3, Bid and Bim. Many of these, plus numerous other proteins, form a complex known as the apoptosome.

The central inducer of cell death is mitochondrial cytochrome c, which is released into the cytoplasm and complexes with Apaf-1 and caspase 9 (the apoptosome) and ultimately leads to caspase 3 and 7 as the final executioners. More recently, mitochondria have been recognized as sensors and regulators of cytosolic Ca 2+ levels, through Ca 2+ transporters which have central physiological roles in signalling, proliferation, metabolism and ultimately cell survival.
There are many ‘death receptors’ (receptors which initiate the cell programme leading to death of the cell, i.e. apoptosis), including FasL, TNFR and granzyme B, as well as environmental conditions which promote cell death, such as hypoxia, genotoxic damage, cytokine or nutrient deprivation (linked to the tricarboxylic acid cycle and cytochrome c) ( Fig. 4-7 ), excess steroid exposure, UV exposure and toxic drug exposure, and the balance between life and death is maintained by pro- and anti-apoptotic Bcl-2 proteins (see Ch. 7 ).

Mitochondria have their own complement of DNA (but no histones, and thus are not susceptible to epigenetic changes; see Fig. 4-1 ) as well as ribosomal RNA/transfer RNA, and generate a series of mitochondrion-specific proteins associated with mutations and a number of discrete syndromes, some with ophthalmological consequences (see Ch. 3 , p. 156 ). Since mitochondria originate only from ova, transmission of these genetic defects is purely maternal.
The nucleus
The nucleus is contained within a perforated sac, formed by pores (nuclear pore complexes). These allow transport of proteins and other signalling molecules passage between the transcriptional machinery and the appropriate instructions from outside. Both import and export of materials are receptor-mediated in an ‘address-label’-like system based on small GTPases (see Box 4-3 ).
The main nuclear component is chromatin, a complex structure of highly extended DNA, RNA and protein in the interphase (non-dividing) cell, which becomes greatly condensed (by 400-fold) to form chromosomes during cell division. Packing of chromatin is achieved by interaction between negatively charged DNA and certain basic proteins (histones) which carry a positive charge at the pH of the cell; euchromatin is less packed than heterochromatin, the proportion of which varies from nucleus to nucleus and may be characteristic of certain cell types, e.g. the ‘clock-face’ heterochromatin of plasma cells detectable in histological sections. Genome stability is dependent on minimizing DNA damage, which might occur for instance during cytokinesis and chromatin condensation, and chromatin regulators exist to ensure stability. Most of these are histone-modifying enzymes such as acetyl and methyl transferases and deacetylases. Much of gene modification is mediated via reversible nucleotide methylation and acetylation.
The nuclear membrane also contains receptors for ligands, which may be synthesized in the cytoplasm or may have been endocytosed through plasma membrane receptors. Typical nuclear membrane receptors include steroids, growth factors such as fibroblast growth factor and novel groups of proteins known as the peroxisome proliferator-activated receptors (PPARs), which are involved in many cell processes such as lipid and glucose homeostasis, wound healing, and inflammation generally. PPARs are unique receptors that allow integration of signals mediated by lipophilic ligands with plasma membrane-derived signals. Nuclear membrane receptors may be organized for induction or suppression of genes in a coordinated fashion ( Fig. 4-8 ). In addition, a major function of the nucleus is to manage the packaging of mRNA into ribonucleoprotein particles called mRNPs. These are associated with very large numbers of proteins involved in the transcription of mRNA in the nucleus to translation and degradation of mRNA in the cytoplasm ( Fig. 4-9 ).


The nucleolus is essentially composed of RNA and fibrillar material, and is the site of ribosomal RNA synthesis and intense transcriptional activity. In addition, it may contain non-coding regions of RNA involved in the stress response. The nucleolus develops during the late stages of mitosis in association with specific regions on the chromosomes, known as the nucleolar organizer centre.
The intracellular matrix
The cytoplasm is a highly viscous aqueous medium that has deformability (elasticity). Physically, it exists at different times as a gel or as a sol. The cortical cytoplasm (ectoplasm) is more akin to a gel structure, while the endoplasm is usually more fluid. Thus the cortical cytoplasm restricts movement of organelles such as coated vesicles (see Box 4-2 ). The gel-like properties of the cytoplasm are the result of the binding of ‘structured’ water molecules and Ca 2+ ions to polymeric filaments; in fact, water, as the main constituent of cells, has a significant role even within the molecular interstices of cell membrane receptors where in its ‘ordered’ state it contributes to the tertiary structure of the molecule as shown by rhodopsin molecules in the photoreceptor disks ( Fig. 4-10 ).

Three main types of intracellular filaments are described, microfilaments, such as actin; intermediate filaments; and group 3 cytoskeletal fibres, such as tubulin and myosin (see Box 4-5 ). More recently, a fourth type has been recognized as a cytoskeletal element, although this family of molecules was discovered over 40 years ago, namely septins.
Microfilaments.
Microfilaments (5–7 nm), such as actin, tropomyosin and troponin, are universal constituents of cells and are involved in almost every cellular activity, including cell motility, contractility, endo- and exocytosis (see Box 4-2 ) and maintaining structural integrity, where they insert into cell adhesion junctional complexes (see eBox 4-2 ).
Intercellular connections or junctions are structures that make cells into tissues and tissues into organs. There are several types, each of which is composed of junction-specific proteins.
Tight junctions – have no detectable ‘space’ between the cell membranes. They are also known as zonulae occludens; they form a barrier to paracellular diffusion of all molecules, including water and ions. They are also involved in regulation of epithelial cell proliferation and differentiation. They occur at such sites as the blood–aqueous barrier of the ciliary body and the blood–retinal barrier at the apex of the RPE cell. They consist of a system of ridges and grooves, as seen by freeze–fracture studies. There are four major classes of tight junction proteins:
- •
transmembrane proteins – occludin, claudin
- •
adaptors – ZO-1, cingulin, MUPPI
- •
transcriptional and post-transcriptional regulators – AP-1
- •
signalling proteins – αPKC, CDK4.
Claudins have unique abilities to selectively permit transport of charged ions and vary from tissue to tissue ( A ).

Desmosomes – form specialized ‘adherens’ junctions of 20 nm width between cells. Two types are described: spot desmosomes (at single site) and belt desmosomes (as a ring round the apex of the cell). The latter are also known as zonulae adherens. Their probable role is mechanical adhesion: cytoplasmic filaments insert into spot (cytokeratin) and belt (actin) forms ( B ). Several proteins are involved in different regions of the desmosome, including desmocollin, desmoglein and desmoplakin, forming a subfamily of cadherins. The cadherins bind to β-catenin and then to actin-based cytoskeletal proteins such as vinculin and α-actinin, ZO-1 and actin itself. The function of tight junctions (above) is dependent on the integrity of the adherens junctions. Hemidesmosomes have a similar plaque formed in this case by bullous pemphigoid antigen (BPAG1).
Gap junctions – so called because there is a 2 nm gap at this adhesion site between cells. Gap junctions occur in the basal regions of epithelial and other cells. They are a highly organized structure composed of ‘connexons’, plasma membrane domains containing connexins, which have a role in permitting the passage of larger ions such as Ca 2+ , and other signalling molecules, so permitting a coordinated response by a group of cells, as in ocular or cardiac muscle ( C ).
Synapse – a specialized form of junction between nerves, or between nerves and muscles, and characterized by synaptic vesicles in the axon terminal and pre- and postsynaptic thickening of the plasma membrane (see Ch. 5 ).
Actin bundles are brought to a highly developed level in muscle cells. Actin occurs in several forms within the cell, depending on its associated protein, e.g. as a fine lattice meshwork or as a sheaf of fibres (stress fibres). Stress fibres are often the most prominent cytoskeletal features in a cell: they not only generate force but they respond to mechanical tension by reinforcement and in some respects are similar to sarcomeres of muscle cells.
The cellular distribution and, particularly, the degree of polymerization of microfilaments are determined by the nature of proteins that bind to them, i.e. the microfilament-associated proteins ( Table 4-1 ). Monomeric soluble actin (G-actin) is converted to gel-phase polymerized fibrils (F-actin) by association with certain proteins. In smooth muscle cells and fibroblasts, filamin assists polymerization; in contrast, in lymphocytes, profilin and thymosin maintain G-actin in the depolymerized state, presumably to facilitate flexibility in cell shape during rapid migration within tissues. The specificity of these actin-binding proteins is remarkable; examples include the ankyrin–spectrin–actin combination, which determines the red cell biconcave shape, and the spectrin–peripherin–actin combination between rod outer segment disks and plasma membrane. Actin polymerization and depolymerization is a highly regulated process requiring addition of actin monomers to one end of the microfilament and removal at the opposite end, each of which has separate K on and K off constants. This process is under the control of actin-depolymerization factor (cofilins) which alter these rate constants as necessary, leading to changes in the twist of the molecule and promoting severance of the actin filament.
Function | Protein | Cell/structure |
---|---|---|
Gelation | Filamin | Smooth muscle/fibroblasts |
Bundling | α-actin | Muscle |
Fimbrin | Microvilli | |
Talin | All cells | |
Severing | Gelsolin | Macrophages |
Villin | Microvilli | |
β-actinin | Skeletal muscle | |
Depolymerizing | Profilin | Lymphocytes |
Thymosin | All cells | |
Actobinin | All cells | |
Membrane-binding | Vinculin | Adhesion sites |
Spectrin | Red cells, photoreceptors | |
Receptor transport | Capping proteins | Leucocytes |
Junctional complex | Radixin | Liver cells |
Regulation of coordinated changes to the actin cytoskeleton is under the control of intracellular enzymes, particularly the Rho family of GTPases (this includes enzymes such as Rho, Rac and Cdc42, the last of which is a cell cycle-related protein). Certain kinases, known as the p-21 activated kinases (PAKs), are involved in regulating some of the diverse changes induced by Rac and Cdc42. PAKs may determine such cellular responses as polarity in epithelial cells and motility in fibroblasts. For example, coordinated functional regulation of the interaction between dynamin, cortactin, actin-binding protein 1, neuronal Wiskott–Aldrich syndrome protein 1 (N-Wasp-1), profilin and actin occurs during the pseudopodial extension and vesicle formation of migrating fibroblasts ( Fig. 4-11 ). Central to this process is the ARP 2/3 complex which is required for actin nucleation. For instance the Arp 2/3 complex is also involved in a relatively recently described family of actin-binding proteins, the coronins, which are involved in leucocyte migration.

Several isoforms and at least three families of actin exist (α, β, γ) and some of these are located in specific parts of the cell, e.g. β-actin in the cell cortex.
Intermediate filaments.
Intermediate filaments (10–12 nm) are coiled α-helices which act as stretchable components of the cytoskeleton scaffold. They occur in cell-, tissue- and differentiation-specific distribution in both cytoplasmic and nuclear compartments of the cell, and are classified into five groups depending on domain and sequence homology. Their major function is to protect cells from mechanical and non-mechanical damage ( Fig. 4-12 ), and gene mutations account for around 30 different diseases in man, mostly related to skin, muscle and nerve dysfunction. In general, intermediate filaments are important for the correct positioning and function of cell organelles such as mitochondria and ER.

There are five classes of cytoplasmic intermediate filaments, often used to characterize cells in tissue culture or tumours, and one class of nuclear intermediate filaments:
- •
keratins – found in epithelial cells; over 50 individual members grouped into two types, I and II, exist as heterodimers
- •
vimentin – found in mesenchymal cells
- •
desmin – interconnects myofibrils of muscle cells, at site of Z disk and M line, thus maintaining their register
- •
glial fibrillary acidic protein (glia)
- •
neurofilaments – e.g. S100 protein in neuroectoderm; connect with microtubules via small projections
- •
lamins – line the inner surface of the nuclear envelope as a fibrous lattice (karyoskeleton); more than five types.

Cellular junctions are highly specialized: desmosomes and hemidesmosomes (‘junctions’ between the cell and basement membrane in epithelia) have some ultrastructural similarity, but at the molecular level there are clear differences between the constituent proteins. Desmosomes are formed by a series of proteins spanning the cell membrane and the intercellular space (desmoglobin, desmoplakin), while hemidesmosomes contain other proteins such as the α 6 β 4 integrin receptor, which binds to laminin in the basement membrane, plus other proteins such as the bullous pemphigoid and the pemphigus antigens.
They may also have a function in the positioning of the nucleus in the cell in a cage-like bundle of fibrils. Intermediate filaments maintain cell homeostasis by dealing with stress in its various guises ( Fig. 4-13 ), and the cell responds in a number of ways such as generating ‘inclusion bodies’, producing new and regenerating intermediate filements, or by reorganizing the filaments into variously sized bundles.

Interactions between intermediate filaments and microfilaments are mediated by plectin, a >500 kDa dumb-bell-shaped protein that can self-associate and in addition can bind at both ends of the hemidesmosomal protein α 6 β 4 integrin and probably to other junctional proteins.
Group 3 cytoskeletal fibres.
A third group of cytoskeletal fibrillar elements also exists but the fibres are less easy to categorize. Several thick filaments occur as part of the cytoskeleton, such as myosin (myosin comprises 25% of cytoplasmic protein in striated muscle) and microtubules. Microtubules are cylindrical structures about 24 nm wide, comprising 13 globular elements composed of the heterodimer (αβ) tubulin. Stable microtubules occur in flagellae and cilia, while labile microtubules are found in structures such as the muscle spindle. Microtubules are involved in movement and cell motility, including intracellular transport as in axoplasmic flow. They are often required to switch very rapidly from an extending to a shrinking fibril, as in processes such as cell division where a multiprotein structure termed the kinetochore engineers chromosome segregation by facilitating chromosome attachment to spindle microtubules. Rapid growth to shrinkage in the microtubule is achieved through GTP to GDP hydrolysis, mediated through the Rho family of small GTPases; this is known as ‘catastrophe’, while the reverse change is termed ‘rescue’ ( Fig. 4-14 ).

These changes occur in any microtubule-related function, including cell movement, and drugs such as colchicine (used in Behçet’s disease) and taxol (proposed as prophylaxis for proliferative vitreoretinopathy) disrupt microtubule organization and inhibit cell motility and cytokinesis.
Group 3 fibres are thus a class of superfine filaments which combine to form an intracellular meshwork in which proteins do not exist in solution as previously surmised, but are attached to and transported along the filaments with other structures such as ‘free’ ribosomes and small vesicles (polysomes). This arrangement has special relevance to highly organized cells such as lens fibres. Transport of molecules along microtubules involves two families of molecular motors, kinesins and dyneins.
Certain discrete cellular structures are composed of microtubules, such as the centriole (the microtubular organizing centre, a cylindrical structure comprising nine groups of triplet microtubules) and the mitotic spindle. Cilia are remarkably constant structures in eukaryotic cells, composed of nine peripheral and two central bundles of three fused microtubules. Movement occurs by sliding of the outer arm of dynein along the core of tubulin.
Septins.
Septins are a family of proteins expressed in all cell types which are integrators of functions of other microfilaments such as the ATPase role of actin and the dynamic functions of microtubules. There are 13 septins in humans, mutations of which are known to cause specific diseases. Septins are important in cells with high migratory functions, such as lymphocytes. In addition, septin 8, which lies on chromosome 5q31, is associated with retinal degeneration. Their main function in the cell appears to be supportive, e.g. as a scaffold for protein recruitment and as an intracellular compartment organizer, and they are called septins for this reason. For example, several septins in one cell combine to form a hexameric filament of around 25 nm long which acts as an intracellular ‘septa’ (barrier to diffusion) ( Fig. 4-15 ).

In conclusion, therefore, there are extensive interconnections between the four filamentous systems orchestrating many fundamental types of cell behaviour. In addition, the cytoplasm also contains several storage products such as glycogen granules, lipid droplets and melanin in melanosomes, often inside endosomes, which, as indicated above, are transported around the cell by MT-associated proteins and made to function by active fibre contraction as in actin-mediated exocytosis (see Box 4-2 ). With age, some cells, such as the RPE cell, accumulate unwanted intracellular bodies such as lipofuscin granules.
Intracellular signalling mechanisms
As stated above, cells respond to external stimuli by means of cell surface receptors, which convert the external stimulus to a series of intracellular signals (second messengers) directed towards specific cellular functions such as protein transcription for growth control or ion-channel gating in neural responses (see Ch. 5 , pp. 288–295 ).
Second messenger systems are based on a network of reactions involving an agonist, a receptor and an interacting set of coupled proteins. Cyclic adenosine monophosphate (cAMP) is the archetypal second messenger, and the result of such a response is the phosphorylation of a regulatory intracellular protein via a kinase, as for instance serine-threonine kinase or tyrosine protein kinases, which demonstrate great substrate specificity. In contrast the phosphatases, such as protein phosphatase-1 (PP1), which usually bring the kinase reaction to a close, are much more widespread in their range of activity and, consequently have many fewer genes expressed.
During the last 30 years, a family of ligand receptors known as WNTs has been shown to have widespread roles in embryonic development, cancer and wound healing. These molecules define the interaction between matrix and cells via intra- and inter-cell signalling and are essential for developmental processes such as patterning. The name WNT derives from the discovery that the int1 (integration gene) and the (Wg) Wingless gene in Drosophila were identical and there are now known to be several Wnt genes with multiple effects on extracellular matrix proteins.
WNT signalling pathways are central to many developmental and cell biological functions ( eFig. 4-5 ), including planar cell polarity (PCP) mediated by Wnt /Frizzled interactions; β-catenin-dependent signalling which is extensively involved in cell–cell interaction and cell adhesion and includes interaction of Wnt with the low-density lipoprotein receptor (LRP)5,6; and Wnt-calcium (Wnt-Ca 2+ ) signalling which is important calcineurin /NFAT signalling and is involved in cell fate and cell migration. This latter pathway is activated in dendritic cells but not macrophages and induces apoptosis in DC (see Ch. 7 , p. 373–380 ).

The extracellular matrix
Cells exist within a structural framework, the extracellular matrix ( Box 4-6 ), which is secreted by the cells such as the myofibroblast during wound healing and consists of several classes of macromolecules, the most abundant of which is collagen (accounting for 30% of total protein in the organism) ( Fig. 4-16 and Video 4-1).
Extracellular matrix proteins appear to be made up of building blocks (‘structural motifs’), which are protein domains with extensive homology to existing protein structures. For instance, three fibronectin domains are reproduced in many other proteins; the epidermal growth factor-like domain also appears in modular form in proteins such as plasminogen; and the arginine–glycine–aspartate (RGD) cell adhesion site is present in many molecules ( A ).
Fibronectin is a dimer containing discrete domains for the attachment of other molecules ( A ). The modular structure of fibronectin shows that it consists of 12 type I modules (rectangles), two type II modules (violet ovals) and 15–17 type III modules (ovals). The alternatively spliced domains IIIB, IIIA and the V region are shown in yellow. Binding domains for fibrin, collagen, cells and heparin are indicated; dimer forms via cysteine pair at the C-terminus (SS).
Laminin is a cross-shaped trimer in which the B1, B2 and A chains form a triple helix in the stem of the cross. Laminin also has several discrete domains for attachment of various molecules. In addition, it contains a cryptic cell adhesion-binding site, which becomes exposed after partial proteolysis ( B ). Fibrillar collagen is organized in tissues by association with smaller non-fibrillar collagens, e.g. type I with type XII collagen, and type II with type IX collagen ( C ).


Genetic and protein sequence analysis of extracellular proteins has shown that, despite the great variety of matrix molecules, extensive sequence homology exists between them with recurring structural motifs ( Box 4-6 ). For instance, the ‘epidermal growth factor’ motif appears to be present in several apparently different extracellular matrix proteins.
Extracellular matrix proteins determine the structural nature of the tissue
Tissues are defined by their extracellular matrix constituents. For instance, the cornea contains type I collagen filaments, which do not form fibrils of diameter greater than 5 nm; this is important in corneal transparency. The vitreous contains an isoform of type IX collagen, which is different in certain characteristics from cartilage type IX collagen.
Collagen fibres are composed of a triple helix of three polypeptide chains, of which there are 46 different types and which assemble into 29 distinct collagen molecules ( Table 4-2 ).
Fibrillar | Anchoring | FACIT ** | Transmembrane | Beaded filament | Network-forming | Multiplexin |
---|---|---|---|---|---|---|
I, II, III, V | VII | IX, XII | XIII | VI, XXVI | IV, VIII, X | XV, XXVI |
XI, XXIV | XIV, XVI | XVII | XXVIII | XXVIII | ||
XXVII | XIX, XX | XXIII | ||||
XXI, XXII | XXV |
** FACIT, Fibril-associated collagen with interrupted triple helices.
At least 22 of these have been detected in the developing and adult eye. The eye and cartilaginous tissues share six types of collagen not found very frequently in other tissues; indeed, the collagen triple helix ( Fig. 4-17 and Box 4-6 ) has been found as a domain of many proteins and it has been suggested that all such proteins should be included in the collagen family. Collagen types are determined by the combination of the three types of chain forming the α helix core. For instance, type I collagen triple helix is made up of two unique α 1 chains and a unique α 2 chain, coded as [α 1 (I)] 2 α 2 (I); type II collagen is composed of three identical unique α 1 chains, [α 1 (II)] 3 . Each collagen therefore has a set of unique chains that make up the triple helix.

In certain collagens, particularly the non-fibrillar collagens such as types IV and IX, the protein is composed of short segments of triple helix (COL1, -2, -3, etc.) interspersed with sections of non-collagenous (NC1, NC2, etc.) protein. These are also sometimes referred to as FACIT collagens (fibril-associated collagens with interrupted triple helices) ( Table 4-2 ). These proteins usually act as bridges or networks for binding other proteins and forming complex protein aggregates such as basement membranes. Collagens in essence are the structural components that hold organs and tissues together and the mechanism by which they form is interesting: the three polypeptide chains form a monomer by self-assembly from a small nucleus in a zipper-like fashion, much in the way that crystallization occurs, and the formation of fibrils from monomers is also entropy driven. Indeed the semi-crystalline packing of collagen fibres, achieved through lysine hydroxylysine cross-links, confers tensile strength to tissues.
A subcategory of collagenous proteins is included as components of transmembrane proteins, including types XIII, XVII, XXIII and XXV ( Table 4-2 ), and remain following cleavage of the soluble fragment of the cytokine or adhesion molecule by enzymes known as sheddases. They exist as homotrimers of a collagen-specific α-chain. Some transmembrane collagen-like molecules occur as specific receptors such as the macrophage scavenger receptors. Sheddases are of several types and include the ADAMTs (proteinases of the adisintegrin and metalloproteinase family), which are involved for instance in the shedding of molecules such as soluble tumour necrosis factor-α-converting enzyme (TACE) from leucocytes (see Ch. 7 ). This general process is important in establishing the soluble regulatory constituents of particular extracellular matrices.
The transmembrane collagen XVII (originally described as bullous pemphigoid antigen 180, BP180) is important in cellular adhesion because it binds α 6 integrin and laminin 5 extracellularly and β 4 integrin, plectin and BP230 intracellularly. Degradation products of collagens, such as the non-fibrillar collagens XVIII and XV, which yield the anti-angiogenic products endostatin and restin after cleavage, have major roles in regulating cell function through inhibition of matrix metalloproteinases.
The eye contains a wide variety of different collagens. For instance, the cornea contains types I, V and VI in the stroma, while types IV and VII are present in the subepithelial layer. In addition, type XVI transmembrane linker collagen has been found in the basal epithelial cell matrix. The iris contains collagen types I, III and IV, while the zonule contains type IV. The lens contains only type IV, while the vitreous contains collagens II, IX and XI, complexed to the extracellular matrix protein fibrillin. This complex has an important role in vitreous matrix organization.
Certain types of collagen are unique to ocular tissue in that their structure has been modified. Examples include type VIII in Desçemet’s membrane, types II and IX in vitreous (both similar but not identical to cartilage collagens) and type III in the distensible tissue of the choroid. Several of the newer collagens contain domains similar to type IX, and it has been suggested that these may represent a subfamily of type IX collagens ( Table 4-2 ). The more recently described types XV, XVIII and XIX are located in basement membranes and may have a role in the formation of blood vessels.
Collagen does not simply fill the space between cells but engages four types of specific receptors on cells: integrins, discoidin domain receptors (DDRs 1 and 2, a subfamily of receptor tyrosine kinases involved in signalling to the cell), glycoprotein VI (GPVI) receptor (on platelets) and leucocyte-associated immunoglobulin-like receptor-1 (LAIR-1). DDRs and integrins are exquisitely specific in the collagen domain to which they bind and their main function is to maintain tissue stability during intra-tissue cell migration, while GPVI and LAIR-1 are involved in haemostasis after tissue injury.
Elastin is the second major insoluble protein of the extracellular matrix. While collagen occurs in all tissues, elastin is present only in deformable tissues such as blood vessel walls, lung parenchyma and the zonule of the lens. Unlike collagen, elastin does not contain any methionine residues and is therefore separable from collagen after digestion with cyanogen bromide. Elastin is formed from soluble tropoelastin monomers, secreted from fibroblasts and smooth muscle cells, which initially self-associate through hydrophobic domains in a process termed co-acervation in vitro which in vivo corresponds to coalescence into spherical globules of 1–6 μm size. This process of self association aligns lysine residues to form cross-links, via allysine intermediates, desmosine and isodesmosine, a process which is facilitated by the elastin co-acervated droplets depositing onto fibrillin microfibrils together with fibulin (see below) ( Fig. 4-18 ). The final fibre is 90% elastin and 10% fibrillin and cross-links are mediated via a set of lysyl oxidase enzymes, mutations in which have been associated with the pseudo-exfoliation syndrome, the commonest cause of secondary glaucoma and a risk factor for successful cataract surgery due to the role of elastin in formation of the zonule. Elastin has little order to its tertiary structure; instead, it forms a random coil, which tends to become more ordered during deformation.

As for collagen, elastin degradation products have a role in regulating cell behaviour in the matrix and under certain circumstances may be pathogenic, e.g. in promoting tumour invasion and angiogenesis. Even smaller molecules derived from a range of matrix proteins (matrikines) have regulatory activity for connective tissue cells; for instance, the tripeptide GHK, which has stimulatory activity for several cell types and promotes collagen biosynthesis and wound healing overall.
Fibrillins are a family of proteins serving as components of extracellular elastin microfibrils that are responsible for the biomechanical properties of tissues. Cysteine-rich glycoproteins, composed of multiple repeats of a Ca 2+ -binding epidermal growth factor-like domain, are secreted in a proform and polymerize extracellularly. They are present in vitreous (see above) and are an important component of the zonule. Fibrillins have a structural role in long-range elasticity of tissues and are a component of elastic fibres (see above). They also have a role in the fine-tuning of growth factor signals such as those involving transforming growth factor-β (TGF-β) and particularly latent TGF-β-binding proteins (LTBP) involved in morphogenesis. Mutations in FN1 cause a dominant form of Marfan syndrome.
Tenascins are extracellular matrix proteins best described as adhesion modulating, matricellular proteins which do not form major fibrillary structures such as collagen or elastin. They are module-built proteins comprising EGF-like repeats, fibronectin-type III repeats and fibrinogen domains ( Fig. 4-19 ). Several forms are described (tenascin-C, -X, -W, etc.) and phenotypes in knockout mice are broadly normal although there may be some subtle defects in wound healing. Tenascin-Y appears to be restricted to neural tissue, while mutations in tenascin-X have been linked to Ehlers–Danlos syndrome, in conjunction with known defects in pro-collagen and elastin biosynthesis. Tenascins are widely distributed and appear to have an anti-adhesive role, particularly antagonizing the effects of fibronectin. Interestingly, fibronectin is found in the anterior lens capsule, while tenascin is found in the posterior capsule.

Laminins are an integral part of basement membranes and have a characteristic hetero trimeric cross-shaped structure composed of an α chain, a β and a γ chain constructed to shape and an α coiled rod (see Box 4.6 and eFig. 4-6 ).
There are 16 isoforms variously made up for one α (α1–α5), 3β (β1–β3) and 3γ (γ1–γ3) chains that are specific for different cell types (e.g. kalinin in epithelial cells) and are named according to their chain components. For instance laminin-332 (i.e. α3, β3 and γ2 chains) is specific for epithelial cells and binds to the α 3 β 1 and α 6 β 4 integrins.
Laminin-332 ( eFig. 4-6 ) is specific to the epithelial basement membrane and is central to epithelial sheets bound to mesenchyme which have to withstand external forces as in the skin and other mucosal layers. Matrix metalloproteinases are involved in post-translational processing of laminins as they incorporate themselves into the basement membrane to form anchoring adhesions and reciprocally, MMPs (MMP-3, -12, -19 and -20) are activated by laminin degradation products to process the γ2 chain and induce epithelial migration during wounding. Laminin in the basement membrane is tightly bound to nidogen (entactin), a protein that mediates the binding of laminin to heparin-containing proteoglycans (heparin sulphate proteoglycan) such as perlecan and syndecan in the basement membrane matrix.

The infrastructure of the basement membranes is composed of type IV collagen in a highly organized lattice network to which the complex of laminin–nidogen is also bound. The proteoglycan then acts as a space-filling molecule in the basement membrane ( Fig. 4-20 ). Cells are bound to the basement membrane via anchoring fibrils containing type VII collagen and transmembrane proteins such as types XI and XVIII collagen.

Most laminins are produced by the cells adhering to the basement membrane in which they are found; interestingly, the laminins of the internal limiting membrane (ILM) of the retina are synthesized by ciliary body and lens cells. ILM laminin and fibronectin are substrates for ocriplasmin, a therapeutic enzyme with potential for use in vitreoretinal disease.
Non-structural proteins
Many other proteins are distributed throughout the extracellular matrix, which, although not having a direct structural role, have important functions in cell–matrix and cell–cell interactions. Many of these proteins are engaged in self-organization with other ECM proteins (see p. 178 ) to form fibrillar networks. Probably the best known of these is fibronectin, a 250 kDa heterodimer that has multiple cell and molecule binding domains (see Box 4-5 ).
Fibronectin. This protein probably initiates the process by binding integrin receptors on the cell surface and entraining the mechanical forces of the cell through transmembrane connections with the dynamic acting cytoskeleton. Matrix assembly is critically dependent on interactions between matrix proteins and the cells which produce them. Such proteins self-organize (see p. 158 ) through specific domains on cell surface protein receptors such as α 5 β 1 integrin which connect to the intracellular cytoskeleton proteins such as actin via linking proteins such as talin and vinculin. Cell contractility then fashions the shape, contour and architecture of the matrix. Once in place, fibronectin then contributes to the assembly of other matrix proteins through its many molecule-specific domains ( eFig. 4-7 ).

For instance, one of the type III fibronectin domains contains the ubiquitous cell adhesion domain Arg-Gly-Asp-Ser (RGDS in single-letter amino acid nomenclature), found in many other proteins. Such domains bind to other proteins which traverse the cell membrane, anchoring the cell to the matrix, including the integrins. Multiple forms of fibronectin occur by alternate splicing. Two major forms exist: plasma fibronectin, secreted by hepatocytes, and cellular fibronectin, secreted by fibroblasts and forming the ECM fibrillar network. Fibronectin may be involved in reverse integrin-mediated cell signalling and, through the actin-binding protein profilin, may regulate stress fibre formation in endothelial cells and fibroblasts. Fibronectin also has high-affinity binding sites for fibrin(ogen), thus promoting incorporation of fibrin into the extracellular matrix during wound healing. Fibrin binds growth factors such as fibroblast growth factor 2 and vascular endothelial cell growth factor, providing sources for angiogenic stimulation.
Thrombospondins ( Fig. 4-19 ) are a group of extracellular matrix proteins that were first identified as platelet release proteins but that are now known to be secreted by endothelial cells and other cell types. Together with SPARC (secreted protein, acidic and rich in cysteine), tenascins and CCN proteins they are considered to be adhesion-modulating proteins. Five forms of thrombospondin exist, two of which appear to be alternately spliced forms of the parent molecule. Like extracellular matrix proteins, thrombospondins are composed of building blocks, each with specific cellular and molecular adhesive properties. Thrombospondins are involved in the regulation of angiogenesis, for instance in preventing angiogenesis associated with ocular inflammatory disease (see Ch. 9 ). In this regard, they form complexes with a ubiquitous plasma protein, histidine-rich glycoprotein (HRG), which has many regulatory functions in wound healing, cell migration and immune cell function. In addition, thrombospondins are better regarded as matricellular proteins that regulate a variety of processes, including cell adhesion and collagen fibrillogenesis. SPARC ( Fig. 4-19 ) is a component of basement membranes but appears to be essential only for normal lens physiology since SPARC-deficient mice develop age-related cataract and lens rupture at 6 months of age but have no other phenotype. Less is known about CCN (for CYR61/CTGF/NOV) proteins ( Fig. 4-19 ), apart from von Willibrand factor, which is involved in haemostasis.
Several other proteins are present in the extracellular matrix, such as proteases (e.g. plasminogen) and their inhibitors (plasminogen activator inhibitor 1 (PAI-1), α 2 -macroglobulin, etc.). PAI-1 is present at high concentrations around various cells in the quiescent state and is considered important in the regulation of cell migration by controlling the level of cell-associated plasminogen activator required to initiate the degradation of basement membrane proteins. Other important proteins include the matrix metalloproteinases (MMPs) and their inhibitors (TIMP-1 to -3). Some of these proteins are secreted by the cells themselves, while others are synthesized predominantly in the liver and reach the extracellular matrix via the plasma.
Some proteins have their action at a distance from the cell, such as fibrillin, a 350 kDa microfibrillar protein that is involved in the assembly of elastin fibrils ( Fig. 4-18 ). Fibrillin is found in the tertiary vitreous and the zonule (see previous section). Mutations in fibrillin are found in Marfan syndrome, a disease of elastic tissue in which dislocation of the lens is a central feature.
Glycosaminoglycans occur in the extracellular matrix bound to core proteins as proteoglycans
Glycosaminoglycans (GAGs) occur in a variety of forms, essentially based on a repeating disaccharide structure ( Box 4-7 ).
Glycosaminoglycans are long chains of repeating disaccharides based on a common structure: ( A ) heparan sulphate; ( B ) chondroitin 4,6-sulphate. Chondroitin 6- and 4,6-sulphates have additional sulphate groups at the appropriate C atoms (*); dermatan sulphate has the GlcA (glycosamino-) residue replaced in variable lengths of the chain by IdoA (iduronic acid). Heparan has many more sulphate groups and a higher content of IdoA than heparin. Keratan sulphate has galactose instead of glucose, and hyaluronic acid has no sulphate groups.

The prototype proteoglycan was described in relation to cartilage, in which a series of proteoglycans are linked to a hyaluronic acid backbone ( Fig. 4-21 ). This, however, is not relevant to ocular proteoglycans; for instance, hyaluronic acid in the vitreous is not associated with other GAG-containing proteoglycans but is linked to collagen type IX, which in this situation is regarded as a proteoglycan. In addition, keratan sulphate proteoglycan occurs in the cornea in the absence of hyaluronan, where it appears to regulate collagen fibril diameter (see below). In other tissues, hyaluronan has both structural and cell-regulatory activities. For instance, two receptors of the LINK protein family, LYVE-1 and stabilin-1, are important in specific functions of lymphoid cells and endothelial cells, respectively. The main cell surface receptor for hyaluronan is CD44 and after internalization hyaluronan may even have some intracellular functions.

The number of proteoglycans that have been described in relation to other cellular functions has increased greatly ( Table 4-3 ). Proteoglycans (PGs) are thus now described in three families of proteins: transmembrane, hyaluronan-binding and collagen fibril-regulating proteoglycans. Transmembrane PGs have a direct role in specific intercellular interactions, hyaluronan-binding PGs function as links for space-filling effects (e.g. in the vitreous cavity), while collagen fibril-regulating PGs have specific roles, e.g. force-generating in tendons, light transmission in the cornea, mechanical support in bone and deformability in heart muscle. In the cornea, four PGs (decorin, lumican, keratocan and mimecan) are the major keratan sulphate moieties that, together with collagens type VI and XII, are essential for maintaining corneal transparency.
Proteoglycan | GAG | Tissue | Function |
---|---|---|---|
Aggrecan | CS, KS | Cartilage | Structural support |
Versican | CS | Fibroblasts | Cell migration, support |
Decorin | CS/DS | Fibroblasts | Fibrillogenesis |
Fibromodulin | KS | ? Keratocytes | Fibrillogenesis |
α 2 (IX) collagen | CS | Vitreous, cartilage | Collagen binding |
Syndecan | CS, HS | Epithelia, fibroblasts | Morphogenesis |
Basement membrane | HS | Basement membrane | Support |
CD44 | CS | Lymphocytes, epithelia, retina | Cell–cell interactions |
Extracellular matrix molecules are intimately involved in cell adhesion
Some proteoglycans such as the syndecans participate constitutively in cellular adhesion and its associated cell signalling, acting as co-receptors with other receptors such as integrins in the receptor cluster, and thus connect through to the cell cytoskeleton ( Fig. 4-22 ). Other proteoglycans interact variably with glycosaminoglycans such as CD44 and neuropilin, both of which are involved in leucocyte–matrix interactions and may be dependent on the state of leucocyte activation.

Cell adhesion is fundamental to many biological processes, such as morphogenesis, development, immune reactions to foreign proteins and many other processes. Cells adhere both to the matrix and to other cells, and usually do so via specific receptors in the cell membrane, i.e. integrins. Integrins are heterodimeric proteins (they have α and β chains, some of which are common to more than one integrin type), and are described in terms of their chain composition, e.g. α 3 β 5 and α 1 β 6 integrins ( Table 4-4 ; see also Ch. 9 and the role of integrin receptors in leucocyte adhesion). Each cell type adheres preferentially to particular extracellular matrix proteins, depending on the type of integrin receptor it happens to express (see Table 4-4 ). In addition, cells may express different integrins at different times, depending on their state of activation, as, for instance, with the (myo)fibroblast during wound healing (see below). Differential adhesion by cells is shown clearly in the lens where anterior epithelial cells use the α 5 β 1 integrin to bind fibronectin, while the equatorial and posterior fibre cells express the α 6 β 1 integrin.
|
Binding of the cell to the matrix via integrin receptors not only has a structural role but also is involved in transmembrane signalling, which may be two-way, i.e. the matrix may modify the behaviour of the cell and the cell may transmit information to other cells via the matrix (inside-out signalling).
This is an exquisitely sensitive system and is tunable, i.e. the integrins can vary their affinity for adhesion to matrix proteins, as directed by cellular inside-out signalling processes. The molecular subtlety is so exquisite that integrins embedded in discoid membrane structures (nanodiscs) are bent and become extended when they bind ligand. Integrins are kept in a loosely bound state by ‘clasps’ on either side to the membrane. The orientation (tilt) of the integrin molecule precisely determines the interaction with ligand such as fibrinogen/fibrin. Intracellular talin mediates inside-out signalling, which is regulated by kindlins under the control of the small signalling molecule Rap1 ( eFig. 4-8 ).

Epithelial and endothelial cells bind via transmembrane complexes to each other and to the basement membrane
Epithelial and endothelial cells rest on a highly organized basement membrane (see above). Binding of epithelial-type cells occurs predominantly via adherens-type junctions and desmosomes/hemidesmosomes, in which several distinct proteins have been identified ( Table 4-5 ). Some of these proteins are members of what is known as the cadherin family of proteins (cell adhesion proteins), a group of transmembrane proteins that regulate intercellular adhesion (see eBox 4-2 ) .
Adherens junction | Desmosome |
---|---|
α, β, γ Catenin | Plakoglobin |
Plakoglobin | Desmoplakin I and II |
Vinculin | Desmoplakin IV |
α Actinin | Desmocollin |
Tenuin | Lamin B-like protein |
Plectin | Plectin |
Radixin | Desmoglein |
These proteins are not only important in the mechanical support of intercellular interactions but also play a role in inter- and intracellular signalling. Gap junctions in particular facilitate and indeed may permit amplification of signalling events which are important in coordinated cell behaviour, as for instance in an epithelial cell sheet.
Mesenchymal cells bind to the matrix via focal adhesion sites
Mesenchymal cells, such as fibroblasts, keratocytes, chondrocytes and stromal cells generally bind to the extracellular matrix via specific focal adhesion sites that contain transmembrane cytoskeletal proteins. Actin stress fibres bind via α-actinin and talin to the cytoplasmic side of the integrin receptor and to vinculin, while the extracellular component of the integrin receptor binds to extracellular matrix proteins such as collagen and fibronectin (see Fig. 4-20 and eFig. 4-8 ) .
Direct reverse signalling takes place through these sites using the signalling protein, focal adhesion kinase, one of the protein tyrosine kinases. Also involved is a further intracellular protein termed VASP (vasodilator-stimulated phosphoprotein) which integrates profilin–actin binding with talin, vinculin, F-actin and the integrin signalling complex. Together with known ion channel-dependent mechanosensors, focal adhesion proteins such as talin act as mechanosensors responding by induced unfolding of their tertiary structures. Similarly, cofilin acts to sever actin filaments in a negative-dependent fashion based on tension in the actin filament at focal adhesion sites. Certain other mesenchymal cells, such as muscle, have specific proteins in their focal adhesion sites such as dystrophin and paxillin, not only at the site of attachment of the extracellular matrix but also at the neuromuscular junction. A mutation in the dystrophin gene has been identified in patients with muscular dystrophy. An integrin-linked kinase appears to be central to regulation of outside-in and inside-out signalling in adherent cells.
Biochemical pathways that affect ocular function
The eye, particularly the outer retina, is highly metabolically active and requires large amounts of ATP, the universal energy storage molecule, for this purpose. Cells cash in on this energy bank by hydrolysing ATP and coupling this event to cell-specific enzymic reactions that are otherwise energetically unfavourable. ATP is generated by oxidative metabolism, particularly of glucose but also of other molecules such as fats and proteins.
Hydrolysis of ATP is not without risk to the cell: free electrons are produced (H + ), which are normally mopped up by nicotinamide adenine dinucleotide (NAD). Indeed, NADH is the main electron carrier in the oxidation of glucose and other molecules, while NADPH (see below) is used to reduce certain molecules such as free fatty acids to permit them to enter the metabolic pathways.
Oxidative consumption of glucose requires coenzyme A, which is a carrier of acyl groups. Other coenzymes are also required for active metabolism, many of which are derived from vitamins.
Glucose metabolism and tissue glycation
Glycolysis is the conversion of glucose to pyruvate in the absence of oxygen, and is accompanied by a net gain of two molecules of ATP. In the presence of oxygen, pyruvate enters the citric acid cycle with the production of 24 molecules of ATP. NAD + is regenerated in the mitochondrial electron transport chain. Glucose and other fuel molecules entering the citric acid cycle may thus be consumed to provide energy for synthesis of the building materials for other molecules such as amino acids.
Not all the fuel molecules are utilized immediately, but instead they are diverted to produce molecules that act as sources of power rather than energy. This is achieved via the pentose phosphate pathway, in which NADPH and ribose are produced, the former for use in reductive processes and the latter in the biosynthesis of nucleotides for RNA and DNA. These different pathways are briefly summarized in Figure 4-23 .

The pentose phosphate pathway also permits glucose formation from unrelated precursors (gluconeogenesis) but does not occur in the brain owing to lack of glucose-6-phosphatase; this probably also applies to the retina. However, the pentose phosphate pathway is active in the cornea and lens (see below).
Finally, glucose may be stored in the liver and muscle as glycogen, whence it can be retrieved for energy purposes by glycogenolysis. The Müller cells in the retina also contain stores of glycogen, which may be essential to maintain retinal function (see Chapter 1 , Chapter 5 ).
Energy metabolism is controlled globally by nutrient intake, use and storage and is regulated by a range of signalling pathways targeting food excess such as insulin, insulin-like growth factor-1 and mammalian target of rapamycin (mTOR); food restriction is likewise controlled by a set of pathways including the ATP-consuming anabolic pathways and ATP-generating catabolic pathways, which are under strict regulatory control by a ‘master-switch’ enzyme system, adenosine monophosphate-activated protein kinase (AMPK), which controls the overall whole body energy metabolism and is involved in loss of neuroprotection in the brain, and possibly the retina, in ischaemic states. In addition, sirtuins, a family of seven histone-deacetylase proteins in mammals, serve as sensors of energy (ATP) levels and also have wider transcriptional and other regulatory roles such as protection from toxicity of reactive oxygen species via upregulation of AMPK.
Glucose enters cells by facilitated diffusion
Glucose transporters (GLUTs 1–14) are members of the SLC2 (solute carrier 2) gene family and are membrane proteins which permit the facilitated diffusion of hexoses and polyols, including myoinositol, glucosamine and ascorbate, into and out of cells down their concentration gradient. Different cells and tissues have different GLUTs, which is relevant to how each tissue handles glucose ( eFigs 4-9 and 4-10 ).
The glucose transporters have a closely defined mechanism for shifting glucose molecules across the cell membrane ( eFig. 4-9 ) and are specific to tissues and cells ( eFig. 4-10 ). Glucose is transported across the membrane in aqueous phase propelled by hydrophobic interactions between glucose molecules and residues on the amino acids in the transporter protein which maintain phase separation of the molecules, while other amino acids are dedicated to promoting binding and thus carriage of the molecule across the glucose gradients that exist. About one-third of the glucose is transported through the gut via Glu2 and Glut 5 (for fructose) and is carried via red blood cells due to their very high concentration of GLUT1. In the resting state most of the glucose is utilized by the brain and nervous tissues while during exercise muscle uses most of the circulating glucose. Adipose tissue regulates glucose utilization via production of hormones termed adipokines.


Thus skeletal muscle utilizes GLUT 4, which is dependent for its function in the plasma membrane on insulin; in contrast, brain and retina, which are considered to be insulin-independent tissues, express GLUTs 1 and 3. The liver and adipose tissue also have GLUTs 2 and 7, with several of the other transporters. GLUTs 1, 3 and 4, particularly GLUT 1, can be upregulated in response to hypoxia via the gene hypoxia-inducible factor-1, which also induces other genes such as vascular endothelial cell growth factor, which is important in retinal vessel permeability and new vessel growth. GLUT 4 in muscle is critical for whole body glucose homeostasis; GLUT 7 is involved in gluconeogenesis and glycogenolysis in the liver, where it is located in the endoplasmic reticulum in association with glucose-6-phosphatase, the essential enzyme for these reactions.
Excessive levels of glucose may impair cellular metabolism
Glucose metabolism is tightly regulated by insulin, IGF-1 and glucagon release from the pancreatic β cells. In diabetes mellitus, in which there is impaired secretion or utilization of insulin, hyperglycaemia leads to excessive uptake of glucose into cells, despite negative feedback on the expression of GLUTs on the cell surface. This has the effect of overloading the metabolic pathways with activation of alternative routes for glucose handling such as the aldose reductase pathway ( Fig. 4-24 ).

In certain tissues, such as the lens, the effect of increased sorbitol may be to cause damage by osmotic dysregulation because sorbitol cannot be transported out of the cell easily. In this case, even high glucose can have direct osmotic damaging effects on the cell owing to raised intracellular [Ca 2+ ] i perhaps as a result of cell shrinkage with consequent activation of the stretch receptors. Alternatively, the excessive utilization of NADPH in this pathway might have deleterious effects on the levels of myoinositol, which is required for intracellular signalling, or on the increased generation of reactive oxygen species directly via activation of the phosphoinositol pathway.
It has therefore been suggested that activation of the aldose reductase pathway is not the direct cause of cellular damage but is merely a coincidental perturbation in the cell; instead, excessive production of free radicals may be important during oxidation of the high concentrations of glucose. Interestingly, aldose reductase has been implicated in inflammatory processes by promoting lipid peroxidation and free radical generation (see below). Whatever the mechanism, aldose reductase is likely to be involved in the pathophysiology of the lens at least, because inhibitors of this enzyme prevent the development of cataracts.
Chronic hyperglycaemia has also been shown to alter cellular metabolism via modulating insulin post-receptor intracellular signalling, involving the cascade insulin receptor substrate 1 (IRS-1)/phosphatidylinositol 3-kinase (PI3K)/Akt, as the result of production of high levels of hexosamine in the cell. This may be one mechanism of induction of insulin resistance in diabetes mellitus. A more general mechanism for the effects of high ambient glucose concentration on cell behaviour has been the induction of the cytokine TGF-β with its widespread effects on cell function, particularly increased proteoglycan synthesis and consequent basement membrane thickening. Patients with type II diabetes are known to have increased serum levels of TGF-β.
Excessive levels of glucose lead to glycation of proteins
Addition of sugar moieties to proteins can occur by enzymatic conjugation (termed glycosylation) or non-enzymatic conjugation (termed glycation). Glycation of proteins is classically considered to occur in the presence of high concentrations of glucose in two phases: an early reversible phase during which the protein forms a Schiff base and Amadori products, followed by a later irreversible phase in which advanced glycation end products (AGEs) appear ( Fig. 4-25 ). However, AGEs can be produced in the early phase because free glucose degrades to α-oxoaldehydes, which are potent glycating agents. AGEs can thus be formed at any stage in the process of glycation, including through binding of Schiff bases and fructosamine. In addition, α-oxoaldehydes such as glyoxal, methyl glyoxal and 3-deoxyglucosone are produced as glycolytic intermediates and also during lipid peroxidation (see below), and lead directly to AGE formation ( Fig. 4-25 ).

Glycation of proteins occurs at lysine, arginine and cysteine amino acids, thus affecting proteins such as collagen (pentosidine cross-links) and haemoglobin and occurs as part of ageing and pathologically in diabetes mellitus; in addition, important extracellular matrix proteins, such as PAI-1 (see above) may be glycated, leading to defective control of cell behaviour, including cell migration and activation. Glycation of cell membrane proteins such as Ca 2+ channels in pericytes may impair their function, thus rendering them less responsive to endothelin-1-induced contractility. The two processes of non-enzymatic glycation and auto-oxidation of proteins are also directly interrelated and may be important in glucose-derived oxidative stress. This last effect is a direct inducer of apoptosis and mediates neuronal and endothelial cell death in diabetic neuropathy and vasculopathy, through binding of AGEs to a specific receptor, RAGE.
A number of enzymes are known to repair proteins in the early stages of glycation, including fructosamine-3-kinase and glyoxalase and the term ‘carbonyl stress’ is used to describe imbalance in glycation versus deglycation.
Interestingly, the free radical scavenger vitamin B 6 may inhibit AGE production via the inhibition of oxidative degradation of Amadori intermediates and the trapping of reactive oxygen products (see below).
Glucose and lipid metabolism
Glucose and lipid metabolism are also intimately linked via the metabolite acetyl coenzyme A. In the presence of excess glucose, production of fatty acids and cholesterol through acetyl coenzyme A is increased with consequent increases in phospholipids (see below) and circulating levels of very low-density lipoproteins. While this has well-known implications for the development of atherosclerosis, more recently this form of metabolic dysfunction has been suggested to underlie pathologies such as age-related macular degeneration, not simply through the production of abnormal lipid deposits in areas where their removal is difficult, such as the subretinal space, but also by a concomitant increase in lipid-based free radicals (see below).
Oxidation/reduction and free radical production
Oxidative metabolism and the generation of ATP storage energy molecules are conducted via the cytochrome enzyme system. However, during the process of oxygen consumption, a small amount of oxygen (<5%) is metabolized by alternative pathways. Univalent reduction of oxygen produces highly reactive free radicals, namely the superoxide anion and the hydroxyl radical, and the toxic molecule hydrogen peroxide ( Fig. 4-26 ).

The superoxide anion is generated in mitochondria in the ubiquinone cytochrome b system and in other membranes by auto-oxidation via the cytochrome P 450 -linked reductases (also part of the drug-metabolizing system), which are rich in the vitamin flavoprotein. Superoxide is then converted by superoxide dismutase to hydrogen peroxide (H 2 O 2 ), which diffuses into the cytosol. Superoxide is also generated in the cytosol itself via recycling of redox enzymes such as xanthine oxidase; conversion to H 2 O 2 in the cytosol is rapid. Xanthine, in particular, accumulates in certain tissues during ischaemia, and subsequent reperfusion leads to massive release of free radicals, producing extensive tissue damage. This can be prevented by free radical scavengers.
Free radicals can be generated by other mechanisms. Reduction of Fe 3+ to Fe 2+ , by reducing agents such as ascorbate, catalyses the conversion of H 2 O 2 to OH • , and auto-oxidation of other compounds such as thiols and catecholamines produces free radicals. The Fe 2+ ion has particular relevance to the problem of retained intraocular metallic foreign bodies. Tissue damage during inflammation is in a large part attributable to the production of free radicals by phagocytic cells during the respiratory burst (see Ch. 7 ). These interactions are illustrated in Box 4-8 .
Free radicals are detoxified by a variety of enzymatic and non-enzymatic mechanisms ( Table 4-6 ). In addition, a critical redox regulatory system involving glutathione and the enzyme glutathione S-transferase is central to cellular homeostasis ( Fig. 4-27 ). Superoxide dismutases require divalent ions such as Mn 2+ , Cu 2+ and/or Zn 2+ for normal activity; some of these have been demonstrated in ocular tissues. Further reduction of H 2 O 2 is either by enzymatic or non-enzymatic means, and may involve several mechanisms directed towards removal of reactive oxygen species (see Box 4-8 ).
Mechanism | Agent |
---|---|
Superoxide anion degrading enzymes | Superoxide dismutases |
Antioxidants | Ascorbate |
Free radical scavengers | Vitamins A and E |
H 2 O 2 degrading enzymes | Catalase, peroxidase |

The mechanism of cell damage varies with each molecular species. Chelated metal ions are important in non-enzymatic degradation of H 2 O 2 , producing the highly reactive OH • radical, which is particularly damaging to cell membranes. H 2 O 2 causes damage by inhibiting glycolysis and glucose uptake and is the most stable of the reactive oxygen species as well as being generated by several intracellular processes (see eFigs 4-10 and 4-11 ).
Moreover, it is likely that free radicals, and in particular H 2 O 2 , involved in cell damage in various ways. They are not only a cause of acute damage and the stress response but also have a cumulative effect that may contribute to ageing. This may be more than simply a by-product of damage: some of the mitochondrial production of H 2 O 2 is generated by an enzyme involved in the apoptosis pathway which induces oxidative stress and may accelerated ageing ( eFig. 4-11 ). Thus the genes that control production may have a say in determining lifespan.

Oxidative stress is considered one of the major mechanisms underlying DNA damage and has been correlated with changes in telomere length associated with ageing. Cells respond to DNA damage using a protein termed protein kinase ataxia telangiectasia mutated (ATM) which mediates DNA repair, cell cycle arrest and apoptosis. Oxidative stress can directly activate dimeric ATM (usually the monomer is the active agent), which seems to act as a cellular sensor for potential oxidative damage.
Lipids and lipid peroxidation
Lipids are the structural basis on which cell membranes are built and are generally composed of a hydrophobic tail of two fatty acid chains bound to a hydrophilic (polar) head ( Box 4-9 ). Fatty acids, thus, have at least three roles:
- •
as integral components of phospholipids and glycolipids
- •
as functional molecules, e.g. hormones and second messengers
- •
as energy stores in the form of triacylglycerols.
The general composition of lipids is a hydrophobic tail consisting of fatty acids bound to a hydrophilic head, which characterizes the molecule ( A ). Glycolipids differ from phospholipids in having a sugar moiety rather than a phosphorylcholine linked to the fatty acid chain. Sphingomyelin is intermediate between these ( B ).


The formation of lipid bilayers is a spontaneous event, due to the physical hydrophobic interactions between the hydrocarbon tails, assisted by polar interactions between the aqueous phase and the hydrophilic head, and between adjacent polar groups. Furthermore, lipid bilayers form closed compartments in which defects are self-sealing, even after disruption. In many membranes, however, proteins, especially receptors and co-receptors, are distributed in clusters while the lipids form lipid rafts which differ in their lipid composition from adjacent regions (see p. 162 ).
Fatty acids undergo physiological degradation during energy consumption by oxidation and polyunsaturated fatty acids (PUFAs) are particularly susceptible. Similarly, lipids in cell membranes can be oxidized. Indeed, peroxidation of lipids is one of the main sources of cell damage, and polyunsaturated fatty acids are particularly susceptible to this form of non-enzymatic damage.
The lipid peroxides oxidize neighbouring fatty acids and set up chain reactions in the membrane. Phospholipase A 2 , by releasing free fatty acids from the membrane, is thought to detoxify this reaction. However, the arachidonate released is then converted to prostaglandins and other eicosanoids ( Box 4-10 ; see also Ch. 7 , p. 382 ). Arachidonates may also be modified by interaction with free radicals in a non-enzymatic reaction. This produces a series of prostanoids, prostaglandin-like chemicals termed isoprostanes. In fact, isoprostanes are produced in much larger quantities than prostaglandins and are detectable in vivo as a measure of lipid peroxidation, and thus indirectly tissue damage. Isoprostanes occur at low levels in normal healthy individuals, thus reflecting a level of oxidative injury not completely suppressed by natural antioxidants. However, as biomarkers of disease hydroxyoctadecadienoic acids (HODEs), hydroxyeicosatetraenoic acids (HETEs), and hydroxycholesterols appear to be sufficiently stable end products of lipid peroxidation, as are isoprostanes and neuroprostanes. Isoprostanes have biological and potentially pathological effects on cell function, thus directly contributing to disease.
Phospholipids, especially those containing arachidonic acid (20:4) and docosohexanoic acid (22:6), a fatty acid abundant in photoreceptor cells, are oxidized to lipid free radicals, which are then conjugated to form dienes. These are then converted to lipid peroxides.
Oxygen flux = D K / L × Δ P
Peroxidation of lipids in cell membranes leads to the release of large quantities of arachidonate, which acts as a substrate for eicosanoid production.

Release of carbonyl compounds from both carbohydrates and lipids can lead to protein modification by glycation (AGEs, see above) and by lipoxidation (ALEs, advanced lipo-oxygenation end products). These are seen especially in uraemic patients on dialysis. Vitamin B 6 (pyridoxamine) may also inhibit ALE production as well as AGE production (see above).
Lipid peroxidation can be limited by vitamin E, a lipid-soluble free radical scavenger that is present within the bilayer (especially photoreceptors) and reacts directly with the lipid free radical to produce the phenoxy radical, or with ascorbic acid in the aqueous phase (see above).
Lipids also occur extracellularly and are of particular importance as lipoprotein carriers in the circulation, and also as lubricants on surfaces. This is particularly important for the ocular surface.
The ocular surface
The ocular surface comprises the conjunctival and corneal non-keratinized epithelium and is bathed by the tear film. However, integrity of the surface depends on other structures such as the apposition of the eyelid margins, the meibomian gland and the lacrimal gland secretions. Collectively, this is termed the lacrimal functional unit (LFU). Evaporation of the tear film is clinically measurable as the tear film break-up time, and tear film integrity is restored by blinking. Blink rate and break-up time are therefore linked. Tears provide lubrication for lid closure, assist in smoothing out the irregularities in the ocular surface (which otherwise may have transient effects on light transmission), and also have an antibacterial function.
The tear film
The tear film is a protective covering for the cornea composed of three layers: a surface oily layer, an aqueous layer and a deep mucous layer. Previous estimates of tear film thickness suggested that the aqueous layer comprised more than 95% of the tear film, but this has been reduced to 60%, with a larger component being provided by the mucous layer based on evidence from in vivo studies using the confocal corneal microscope. In addition, clinical evaluation of the tear film involves an assessment of the tear meniscus height (TMH). The TMH reflects the volume of tears that collects at the contact line between the eyelid margin and the bulbar conjunctiva and has several other names, including the inferior marginal strip and the tear prism or rivus.
The cornea presents a hydrophobic non-wettable surface, which is made wettable by possessing a layer of mucus on its surface. This is overlaid by the aqueous component of the tear film and lastly by a layer of lipid, which prevents evaporation of the tears ( Fig. 4-29 ). Soluble mucus in the aqueous layer and meibomian lipid combine to ensure stability of the tear film by lowering the surface tension and permitting spreading of the tear film on blinking. The break-up time is therefore thought to represent the time it takes for aqueous to evaporate and meibomian lipid to come in contact with the hydrophobic epithelial cell layer.

The blink reflex removes up to 70% of the surface aqueous–mucous layer while the remaining 30–40% thick mucous layer in contact with the cornea is not wiped away on blinking. Instead, it is thinned by blinking and may take 30 minutes to reconstitute itself. The mucous layer is in part composed of the glycocalyx of the epithelial cells and also by an additional layer of tear mucins produced by the conjunctival goblet cells. Reduced tear film break-up time in dry eye disorders of various types therefore reflects a disturbance in tear mucin/aqueous protein interaction and may be associated with a reduction in goblet cell density.
Tear film lipids have unique characteristics
The lipid layer of the tear film is derived from meibomian gland secretions and is very thin (0.1 µm). It is composed of a mixture of polar and neutral lipids ( Box 4-9 ) with a melting point (35°C) that ensures it is always fluid on the ocular surface. The polar lipids are in contact with the aqueous phase of the tear film and provide structural stability to the tear film, while the non-polar lipids are at the air interface and provide barrier function and thixotropic properties. The lipids include unsaturated and branched-chain fatty acids and alcohols, 8–32 carbon chains in length. Tear lipids are under study by way of lipidomics and different sets of wax esters, oleates and cholesterol esters have been identified. Interestingly, in comparison with lung surfactant, there is a more heterogeneous composition with prominence of wax esters ( Fig. 4-30 ).

Tear lipids promote movement of water into the aqueous phase during formation of the tear film, where they bond to tear lipocalins. The functions of the meibomian lipid layer are:
- •
to prevent evaporation of tears
- •
to prevent spillover of tears at the lid margin
- •
to prevent migration of skin lipid onto the ocular surface
- •
to provide a clear optical medium.
Lacrimal gland secretion provides the aqueous component of tears
The lacrimal gland and its accessory glands (see Ch. 1 , p. 89 ) are classic exocrine acinar glands secreting a dilute aqueous solution containing proteins and small molecular weight components and electrolytes ( Table 4-7 ). Although over 1500 proteins have been identified in tear fluid by preoteome analysis, the principal proteins in tears are immunoglobulin A (IgA), lactoferrin, G protein, tear-specific prealbumin (lipocalin) and lysozyme ( Fig. 4-31 ). Tear-specific prealbumin is a member of the lipocalin superfamily and together with a further lipocalin, apolipoprotein D, is secreted by the lacrimal gland. The function of tear lipocalin is to interact with meibomian gland lipid and induce surface lipid spreading. It may also have a role in removing harmful lipophilic molecules.
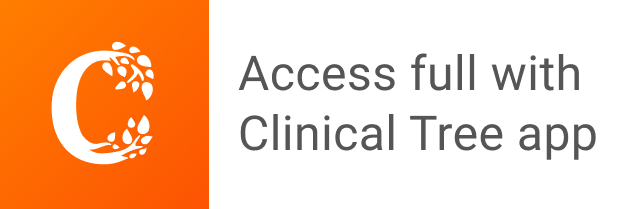