Introduction
This chapter aims to provide an embryological basis for understanding the anatomy of the eye and adnexa. Basic embryological and developmental events from the earliest formation of diverticula in the forebrain at the beginning of the fourth week to the maturation of the various components of the eye in fetal life that influence the final differentiation and functional specialization of the adult eye are described. The contribution of the neural ectoderm, surface ectoderm and periocular mesenchyme to the final configuration of the adult eye and surrounding tissues of the head is emphasized. The cellular interaction and influence of gene expression and transcription factors in determining cell fate will be outlined and examples are given where disruption of gene function underlies disturbances in these embryological events and the interactive processes between the various embryonic tissue and cell types lead to congenital abnormalities in humans.
General embryology
Before considering eye development, which commences in the fourth week, it may be useful for some readers to review the embryological events of the first 3 weeks following fertilization (see eFig. 2-1 and Video 2-1).

The male and female gametes unite at fertilization, which generally occurs within the oviduct or uterine tube, to form the zygote. The newly formed diploid cell undergoes cleavage as it travels towards the uterus. Within the solid mass of cells (30-cell stage) or morula , the blastocyst cavity appears. The earliest differentiation of the embryo occurs around this stage when two groups of cells are formed: a peripheral outer cell mass or trophoblast and a central inner cell mass or embryoblast. The embryoblast will give rise to the embryo proper and some of its attached membranes. By day 5 or 6 the embryo is a hollow spheroid of around 100 cells, known as the blastocyst. The embryoblast is evident as a heterogeneous collection of cells at one pole of the blastocyst; the remainder forms the trophoblast. This differentiates into the syncytiotrophoblast and the cytotrophoblast, which contribute to the fetal component of the placenta. Around this time the blastocyst enters the uterus and commences implantation into its rich endometrial lining.
At the beginning of the second week two cavities appear within the embryoblast. One forms the amniotic cavity , lined by epiblast cells, while the other forms the yolk sac , lined by cells derived from the hypoblast. Where the two cavities impinge there is a double discoid layer of cells, the epiblast or primary ectoderm and the hypoblast or primary endoderm. These two flat disks of cells constitute the bilaminar germ disk , which will develop into the embryo proper.
The third week of development commences with formation of the primitive streak and primitive knot or node at the caudal (tail) end of the epiblast. It is here that epiblast cells detach and migrate laterally and cranially into the potential space between the epiblast and the hypoblast to form the intraembryonic mesoderm or third germ layer. Some of the epiblast cells also replace the original hypoblast to form the definitive or secondary hypoblast. The epiblast is now known as the ectoderm. Formation of the primitive streak establishes the craniocaudal axis and bilateral symmetry of the future embryo. The formation of the notochord by budding from the primitive knot induces the formation of the neural plate. A series of paraxial mesodermal condensations or somites form along each side of the neural plate as it folds to form the neural tube , the precursor of the central nervous system ( eFig. 2-1 ). A special group of cells, the neural crest cells, detach or delaminate from the margins of the neural folds and undergo extensive migration throughout the embryo where they differentiate into a remarkable variety of cells and tissues ( eFig. 2-2 ). Derivatives of trunk neural crest cells are shown in eFigure 2-2 . Derivatives in the head are more extensive.

Anatomically, the human embryo is described as being in the prone (face-down) position. The terms dorsal and ventral correspond to posterior and anterior in the adult. The terms rostral and caudal correspond to superior (head end) and inferior (tail end).
Ocular embryology: general introduction
Although eye development can be considered from an embryological perspective to commence around day 22, when the optic sulci (optic primordium) appear as shallow grooves or pits in the inner aspect of the neural plate or neural folds ( Fig. 2-1A ) and the embryo is around 2 mm in length with eight somites, the group of cells that constitute the eye primordium or eye field have already begun to express a set of ‘eye field transcription factors’ (EFTFs) that are highly conserved in our evolutionary ancestory (see below). The neural folds have commenced fusion to form the neural tube but the optic sulci form before they have completed their closure rostrally and caudally. When the folds in this area fuse shortly afterwards they give rise to the future diencephalon region of the prosencephalic (forebrain) vesicle. The optic sulci evaginate to form hollow diverticulae – the optic vesicles ( Fig. 2-1B ).


By about day 25 (20-somite stage) the hollow optic vesicles enlarge and become ensheathed by mesenchymal cells, except at the apex of the vesicle, which is closely apposed to the surface ectoderm on the lateral aspect of the developing head. This mesenchyme is derived from a mixture of cephalic neural crest and both para-axial and prechordal mesoderm (see eBox 2-1 ) .
This is a term used to describe the tissue occupying the embryo between the surface ectoderm (and derivatives such as neuroectoderm) and the endoderm-derived epithelial layers. It is a loose tissue consisting of stellate amoeboid mesenchymal cells embedded in a matrix rich in glycosaminoglycans. Mesenchymal cells may be derived from several sources, namely mesoderm (dermatome or sclerotome component of the somites or lateral plate mesoderm) or neural crest. Thus, the descriptive term ‘mesenchyme’ does not imply an origin from any particular embryonic germ layer.
A disk-shaped thickening of the neural ectoderm, the retinal disk (future neural retina), lies beneath a localized thickening of the surface ectoderm, which on day 27 is recognizable as the lens placode ( Fig. 2-1B ). Formation of the lens placode directly adjacent to the underlying neural ectoderm is regarded as one of the best examples of induction in developmental biology. Lens placode formation coincides with the formation of a constriction in the optic vesicle at its attachment to the wall of the forebrain to form the optic stalk. The cavity of the optic vesicle or optic ventricle (future subretinal space) is continuous, via the lumen in the optic stalk, with the future third ventricle ( Figs 2-1B and 2-2A ).

The single-layered spheroidal optic vesicle undergoes active invagination to fold in on itself creating two ‘nested’ layers of the goblet-shaped optic cup. The distal part of the optic vesicle becomes the inner layer or presumptive neural retina, whereas the proximal part of the optic vesicle becomes the outer layer of the optic cup, namely the presumptive retinal pigment epithelium. The thickened retinal disk at the tip or distal part of the vesicle ( Fig. 2-1B,C ) and the ectoderm-derived lens placode invaginate via a combination of differential growth (cell elongation and mitosis) and buckling to form the dorsal hemisphere of the optic cup and the lens vesicle. This combined invagination may also be aided by temporary fine cellular bridges between the lens placode and the retinal disk and is mediated by by the extracellular matrix protein fibronectin-1 ( Fn1 ), whose extent and circumferential limit beneath the lens placode is regulated by Pax6 expression ( Fig. 2-3 ). The active growth of the optic cup is not uniform around the circumference, which leads to the development of a groove at the distal and ventral aspect where the margins form the choroidal or optic fissure. By day 29 invagination of the retinal disk and lens placode is almost complete ( Figs 2-1C and 2-2A ). A small lens pit can be identified just before the surface ectoderm seals over the site of lens placode invagination. By the start of day 36 the lens vesicle separates from the surface ectoderm. The lens epithelial cells enclose the lens cavity and are surrounded externally by a basal lamina, which will form the future lens capsule. The longitudinal groove of the optic fissure, which extends into the optic stalk, acts as a temporary deficiency in the expanding and invaginating cup through which vascular mesenchyme and a branch of the ophthalmic artery, the hyaloid artery , become incorporated into the fissure and thus gain access to the lentoretinal space. By the end of the sixth week the growing edges of the choroidal or optic fissure meet and fuse; thus the hyaloid vessels and associated mesenchyme become situated in the centre of the optic stalk and form the future central retinal artery and vein ( Fig. 2-1D,E ). The fusion or closure of the optic fissure commences at the mid-portion of the optic stalk and continues both proximally and distally. It is completed distally at the margins of the optic cup that will eventually form the pupil.
The word coloboma (‘a mutilation’) in clinical practice is the term is used to describe a defect in the inferonasal quadrant in the iris, ciliary body or choroid. Colobomas are usually sporadic and bilateral and do not lead to significant complications. They are the result of a failure of closure of the (inferonasal) optic fissure. The consequence is an interference with the normal induction and formation of uveal tissues. A coloboma of the iris appears as an inferonasal defect in the stroma, the smooth muscle and the pigment epithelium whereas a ciliary body coloboma is characterized by absence of ciliary processes and presence of a diminutive muscle. The adjacent lens is indented, owing to a failure of formation of the zonular fibres. In the colobomas associated with complex malformations and abnormalities (e.g. trisomy) there may be an ingrowth of mesodermal tissue into the retrolental space with formation of fat and cartilage.
Colobomas involving the retina may be large and may extend to the disk. In the retina adjacent to the coloboma, proliferation of neuroblastic tissue leads to the formation of rosettes. The retinal pigment epithelium and Bruch’s membrane and choroidal tissue are absent at the site of the coloboma, although the underlying sclera is normal. In many cases of retinal coloboma there is glial and vascular ingrowth from the retina across the bed of the coloboma. Colobomas have been described in association with mutations in the CHD7 gene (see Table 2-1 ). Mutations in the CHD7 gene are found in approximately 60–65% of individuals with CHARGE syndrome ( C oloboma, H eart disease, A tresia choanae, R etardation of growth and/or development, G enital hypoplasia, E ar malformation). Most mutations lead to the production of an abnormally short, non-functional CHD7 protein, which appears to disrupt chromatin remodelling and the regulation of gene expression.


Mouse gene | Human gene | Expression pattern | Ocular defects in mouse mutants | Ocular defects in humans with known mutations in related gene |
---|---|---|---|---|
Sox1, Sox 2, Sox3 | SOX1, SOX2 SOX3 | Central nervous system, sensory placodes, Sox2 in anterior neural ectoderm and lens placode | Micro-ophthalmia, cataract | Anophthalmia |
Otx2 | OTX2 | Anterior neural ectoderm Future forebrain | Range of phenotypes from bilateral anophthalmia to retinal dystrophy | |
Rax | RAX | Anterior neural plate, optic vesicle, developing retina, photoreceptors | Eyeless | Anophthalmia |
Pax-6 | PAX6 | Anterior neural plate, optic sulcus/cup and stalk Surface ectoderm (future lens and corneal/conjunctival epithelium) Weakly expressed in mesenchymal cells | Micro-ophthalmia, cataract, hypoplastic iris, incomplete separation of cornea and iris, corneal defects | Anophthalmia, anterior segment dysgenesis, congenital glaucoma, Peters’ anomaly, Axenfeld–Rieger syndrome (aniridia) |
Six3 | SIX3 | Presumptive eye field | Lack of neural retina | Holoprosencephaly |
Pitx3 | PITX3 | Developing lens vesicle | Persistent lens stalk, malformed lens | Congenital cataract, leucoma, Peters’ anomaly |
Chd7 | CHD7 | Neuroectoderm, lens vesicle | CHARGE-like features, keratoconjunctivitis sicca | CHARGE syndrome (see Box 2-1 ) |
Maf | MAF | Lens placode, lens vesicle, primary lens fibres (transcription factors for α-crystallin gene along with Sox1 ) | Failure of lens fibres to elongate, lens vesicle fails to separate from surface ectoderm | Defects in lens, cornea and iris (coloboma), Peters’ anomaly |
Foxe3 | FOXE3 | Lens placode | Failure of lens to separate from surface ectoderm | Peters’ anomaly, posterior embryotoxon, cataract |
Pitx2 Fox1 | PITX2 FOXC1 | Periocular mesenchyme (presumptive cornea, eyelids, trabecular meshwork, extraocular muscle) | Anterior segment abnormalities | Iridogoniodysgenesis, Axenfeld–Rieger syndrome, 50% develop juvenile glaucoma |
Crya, Cryb, Cryg | CRYA, CRYB, CRYG | Lens | Various forms of cataract | Various forms of cataract |

By the end of the embryonic period (defined as the end of week 8 in humans) the retina can be clearly differentiated into a thin outer layer, which will form the retinal pigment epithelium (RPE) and a much thicker inner neural retina ( Figs 2-1F and 2-2F ). These two layers are separated by a narrow intraretinal or subretinal space , the remains of the almost obliterated ventricular cavity of the optic vesicle. Melanin first appears in the RPE around 5 weeks ( Fig. 2-2C,D ) and is visible on external examination of embryos of this gestational age. The neural retina commences its centrifugal differentiation into an inner and outer neuroblastic layer near its continuity with the optic stalk. The lens cavity disappears as the posterior cells elongate to form the primary lens fibres ( Figs 2-1D and 2-2D ). Mesenchyme, mostly derived from neural crest cells, condenses around the external surface of the optic cup. The innermost layer of this mesenchyme is loose and highly vascular and will form the choroid (uveocapillary lamina) ( Fig. 2-2C,D ). It lies adjacent to a distinct basement membrane formed by the RPE, which is continuous with a similar membrane around the forebrain. Indeed, the choroid is homologous in its embryonic origin with the pia mater and arachnoid investing the brain. The outer layer of the condensed mesenchyme will form the sclera , which is homologous to, and indeed continuous with, the dura mater around the optic nerve and brain posteriorly.
In the eighth and last week of the embryonic period ( Figs 2-1F and 2-2F ), ganglion cell axons grow from the inner retina towards the optic stalk. The axons travel within the stalk towards the brain, thus forming the optic nerve. Other major landmarks in development occurring in the eighth week include formation of secondary lens fibres, lens sutures and the secondary vitreous.
In summary, by the end of the embryonic period the eye comprises a double-layered neural ectoderm-derived optic cup containing a surface ectoderm-derived lens, both enveloped by condensed mesenchyme comprising a dense outer layer (the bulk of the future cornea and sclera) and an inner vascular layer which will form the choroid and stroma of the iris and ciliary body. At this stage the human embryo is 30 mm in length (crown–rump length) and the developing eye is 1.5–2.0 mm in diameter ( Figs 2-1F and 2-2F ).
Genetic regulation of eye development
It is now clear that epigenetic development , the process by which invertebrates and vertebrates develop their definitive characteristics through the gradual alteration of simpler precursors, is regulated by cascades of gene expression. That is, early acting regulatory genes initiate developmental processes and induce the expression of ‘downstream’ genes , which may subsequently lead to expression of further genes and so on until genes encoding actual structural and functional characteristics of specific cells and tissues are activated. There is overwhelming evidence that these cascades have been conserved throughout evolution from insects to fish to mammals. The Drosophila , or fruit fly, zebra fish and mouse are the most intensively studied experimental species in each respective group and recent research has shed light on some of the critical genes in ocular development, some of which have been remarkably conserved in evolution. The clues from animal studies have aided clinical geneticists to screen the DNA from patients with abnormalities and have helped reveal how mutations in single genes can cause congenital abnormalities, and the eye has provided science with some of the more elegant examples of the relevance of such genetic studies ( Table 2-1 ) (see Ch. 3 ).
The basic body plan of all animal embryos is initially established by a class of regulatory genes called selector or switch genes which, like the maternal effect genes of the fruit fly, establish longitudinal or antero-posterior (head–tail), dorsoventral and left–right axes. A further class of genes, the zygotic genes , which includes segmentation genes , is switched on later, after the maternal effect genes. The changes induced by the segmentation genes cause the expression of another class of selector genes, the homeotic genes , which encode a region of DNA called a homeobox (termed Hox genes). These subsequently regulate many downstream genes and thus act as master control genes.
Hox gene activation plays a critical role in the differentiation of what initially appear as identical segments in the embryo (induced by earlier expression of segmentation genes) into, for example, cervical, thoracic, abdominal and sacral regions as well as the segmentation of the head and neck, particularly the pharyngeal arches and subdivision of the brain.
Paired-box (Pax) genes encode transcription factors involved in the regulation of several aspects of vertebrate and non-vertebrate early development and, as such, are also considered as ‘master control genes’. In vertebrates two Pax genes, Pax-6 and Pax-2 , are important eye field transcription factors.
Eye field transcription factors
In mammals (and one must appreciate that much of this research is performed in mice) the eye field transcription factors (EFTFs) include Pax-6, Rax, Six3 and Lhx2 and these are expressed in an overlapping fashion in the anterior neural plate or future forebrain at the paired sites that define the site of the eye primordium or eye field ( Fig. 2-4 ). There is evidence that Otx2 , a transcription factor essential for forebrain development, may cooperate with SOX2 , a neural ectoderm transcription factor, to progressively activate Rax expression in the eye field, which may in turn activate Pax-6, Rax, Six3 and Lhx2 . Evidence of the importance of these genetic transcription factors in early developmental events can be seen by considering the effects of mutations in both mouse models and humans (see Table 2.1 ).

The primordial eye field is initially one region of anterior neural ectoderm that has two hemispheres that later split into two respective fields, a left and a right, and at least two molecules ( sonic hedgehog (Shh) and Six3 ) are involved in this early morphogenesis, which, if disrupted, leads to a failure in the splitting, and thus to cyclopia or a single midline eye.
Pax-6 is expressed in the anterior neuroepithelium and lens placode-forming epithelium. Thus it appears that the gene defines a field of cells competent to differentiate into eye tissues (neural retina, retinal pigment epithelium, iris epithelium, ciliary body epithelium, lens and corneal epithelium). It also appears to be required to maintain growth and proliferation of cells in the optic vesicle (and other regions of the central nervous system). Mice with mutations in Lhx2 can generate optic vesicles but never form optic cups and it appears as if both this transcription factor and Pax-6 are needed to form a proper eye cup and together induce Six6 in the optic vesicle, which is essential for retinal differentiation. The boundaries between the parts of the optic vesicle that ultimately differentiate into neural retina, RPE and optic stalk are predetermined by the differential expression of cell signalling pathways along the dorsoventral and proximal–distal axes, which are in turn regulated by transcription factors ( Fig. 2-4 ). For example, a mutually antagonistic relationship exists between expression of Pax-2 and Pax-6 . Pax-2 is largely restricted to the future optic stalks on the ventral aspect where it appears to be necessary for successful closure of the choroid fissure, whereas Pax-6 is expressed more dorsally and is more involved with formation of the RPE and neural retina. These cell-intrinsic signalling pathways are not the only factors that determine the fate of cells or patterning in the optic vesicle. There are further extrinsic factors including members of the transforming growth factor-β (TGF-β), fibroblast growth factor (FGF) and Wnt families and Shh that signal to functionally compartmentalize the optic vesicle. For example, ectopic expression of FGF9 in the presumptive RPE promotes neural retina formation and inactivation of canonical Wnt signalling in the future RPE causes it to transdifferentiate into neural retina.
These occur in the first month of embryonic life and include:
- •
Anophthalmia. Extremely rare and the result of a failure of formation of the optic vesicle. The orbits do not contain ocular tissue, but the extraocular muscles (mesoderm) and lacrimal gland (ectoderm) are present. Mutations in the RAX and SOX2 genes have been associated with anophthalmia ( Table 2-1 ).
- •
Nanophthalmia and microphthalmia Formation of the optic vesicle without proper subsequent development produces a rudimentary eye in the orbit – nanophthalmia (or dwarf eye). In microphthalmia there is a small but recognizable eye that contains recognizable elements, e.g. lens, choroid and retina. The mouse mutant small eye ( Sey ) has a haploinsufficiency in Pax-6 . Human mutations in one copy of PAX6 have aniridia (iris abnormalities) and microphthalmia but homozygous loss causes anophthalmia. Mutations in SOX2 can also cause microphthalmia.
- •
Cyclopia Mutations in SHH or SIX3 result in midline defects including cyclopia.
- •
Synophthalmia. Fusion of the two eyes may result from a malformation of the mesenchymal tissue between the optic vesicles or faulty inductive processes. Only rarely is a single eye (cyclops) formed by this mechanism and in most cases there are two recognizable corneas and lenses, and identifiable parts of the iris and ciliary body. The midline sclera and uveal tissue may be absent and the optic nerve may be single or duplicate. This malformation may be associated with a deletion of chromosome 18.
Periocular mesenchyme is derived from a mixture of neural crest and mesoderm
For many years it was widely held that the middle germ layer, the mesoderm , gave rise to most of the mesenchyme and its derivatives in the head and neck region in a similar manner to the pattern of differentiation in the trunk (see eFig. 2-2 ) .
A large body of experimental evidence from avian and mammalian studies has now shown that mesenchyme in the head region is derived from two sources, neural crest ( mesectoderm ) and mesoderm. Neural crest cells originate at the neuroectoderm–surface ectoderm junction of the fore-, mid- and hindbrain regions before fusion of the neural folds. They migrate ventrally into the pharyngeal arches and rostrally around the forebrain and developing optic cup and into the facial region in a highly ordered manner, guided in their migration by components of the extracellular matrix, such as fibronectin and glycosaminoglycans ( Fig. 2-5 ). The pattern of appearance and migration of cranial neural crest is closely associated with the expression products of the homeobox ( Hox ) gene family within the rhombomeres of the hindbrain. In the face region, neural crest cells contribute significantly to mesenchyme-derived tissues, such as bone, cartilage, connective tissues, meninges and ocular and periocular connective tissues (which normally do not arise from trunk neural crest), as well as giving rise to the usual crest derivatives: melanocytes, dorsal root ganglia equivalents (sensory ganglia of V, VII, IX and X) and parasympathetic ganglia (ciliary, otic, pterygopalatine and submandibular).

At the same time as cephalic neural crest cell migration commences, the optic stalks begin to constrict, thus creating a pathway between the stalk and surface ectoderm into which predominantly mesencephalic crest cells migrate. The migration of these cells ceases when they reach the choroid fissure on the ventral aspect of the optic cup (for further information see eBox 2-2 ) .
The fate of neural crest cells from various regions of the neural plate has been mapped in birds (for reviews, see ; ); it has also been mapped in mouse and rat embryos ( ; ; ). Avian experiments have used a variety of cell-tracing methods, including transplanted radiolabelled donor crest cells, chick–quail transplant chimeras and subsequent tracking of quail cells using natural nucleolus marker or anti-quail nuclear antigenic determinant (for review, see ).
Tracking the fate of neural crest cells in mammals has been aided by the production of mouse mutants with neural developmental anomalies and more recently by utilizing transgenic mice in which a transgene (bacterial lac Z ‘reporter’ gene which codes for β-galactosidase) is introduced into the mouse genome in a position where it will be co-expressed alongside proteins specific for the cell types under investigation, such as peripherin or retinoic acid receptor, which are expressed on neural crest cells during and after migration. The migration pathways of the cells carrying the transfected genome can thus be visualized with appropriate chemical substrates for bacterial β-galactosidase ( ). Other techniques that are unveiling the destiny and differentiation of various cell types in embryonic development include micromanipulative cell grafting and labelling using fluorescent cell markers (Dil and DiO), in situ hybridization and chimeric and mosaic mouse models ( ; ; ) (see Fig. 2-6 ).
Paraxial mesoderm (somites and the less distinct rostrally situated somitomeres ) forms most of the walls and floor of the brain case, all voluntary muscles of the craniofacial region (including extraocular muscles), all vascular endothelial cells, the dermis and connective tissues of the dorsal region of the head, and the meninges caudal to the prosencephalon. The seven rostrally situated somitomeres differentiate in a segmental manner within the pharyngeal arches and they play an important role in eye development by influencing neural crest cell migration and differentiation and by directly contributing to the periocular mesenchyme ( Fig 2-6 ).

Thus, mesodermally derived mesenchyme contributes more to the periocular connective tissues than experimental studies in birds had previously suggested.
The neural retina and retinal pigment epithelium are derived from neuroepithelium
The thickened portion of the optic vesicle that invaginates, the retinal disk , is destined to differentiate into the neural retina , while the thinner outermost layer of the optic vesicle is destined to form the RPE. These layers are continuous at the optic cup margin, where a sharp transition in morphology is evident ( Figs 2-1B,C and 2-2A–D ). The optic cup margin will later be the site from which the neuroepithelial component of the iris and ciliary body will arise and ultimately form the pupil margin. Because of the invagination of the optic cup the apical aspect of the primitive neural retina comes to lie adjacent to the apical surface of the RPE, thereby obliterating the intraretinal space ( Fig. 2-1C ). Just as the ependymal cells that line the developing (and adult) ventricular spaces in the brain are ciliated, the apposing surfaces of the primitive neural retina and future RPE are also ciliated. The cilia of the neural retina are important later in development, in the formation of rods and cones. The cilia of the RPE degenerate.
Axes in the neural retina
We now understand more clearly how the axes in the retina which are critical to establishing the pattern of retinotopic projection become organized during development. Mouse studies have shown that when the inner layer of the optic cup invaginates to form the neural retina it must establish three primary axes: dorsoventral, nasal–temporal and anterior–posterior. In addition, the optic stalk must form inferiorly relative to these axes ( Fig. 2-7 ). The signalling that is important in the dorsoventral axes are mediated through the Pax-6 (highest expression dorsally) and Pax-2 (highest expression ventrally) transcription factors in addition to the VAX family of homeodomain transcription factors. Vax2 , in particular, has a steep ventral–dorsal gradient, wheras Vax 1 is expressed on the optic stalk. Naso-temporal patterning is regulated by the forkhead transcription factors FOXD1 and FOXG1 ( Fig. 2-7 ).

Retinal morphogenesis ( Fig. 2-8 )
The primitive neural retina consists of an outer nuclear zone and an inner acellular or marginal zone. The outer nuclear zone is homologous with the proliferative neuroepithelium of the neural tube. Both the inner and outer layers of the optic cup rest on their respective basal laminae: that of the inner layer becomes the inner limiting membrane , the outer is incorporated into Bruch’s membrane. Differentiation of the retinal layers commences at the posterior pole and progresses in a centrifugal manner; thus a gradient of retinal differentiation can be seen within an individual eye. Miotic activity in the primitive neural retina is greatest in the outer part of the nuclear zone ( Fig. 2-8A ). Around 7 weeks of gestation (16–20 mm) newly formed cells migrate in a vitread direction into the marginal zone to form the inner neuroblastic layer. The outer nucleated zone is now referred to as the outer neuroblastic layer ( Figs 2-8B and 2-9A ). The two neuroblastic layers are separated by an acellular zone – the transient layer of Chievitz. The earliest differentiated cells, which form the inner neuroblastic layer, are future ganglion cells, Müller cells (radial glial) and amacrine cells. Elegant studies using chimeras and mosaic mouse models have revealed that clones of cells radiate in a vitread direction and, as the retina begins to stratify, proliferation and differentiation cells that have arisen from these original ‘clones’ appear as columns. Subsequently some cells disperse laterally.
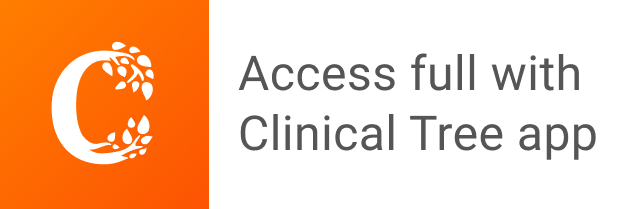