Entoptic Imagery and Afterimages
Gary L. Trick
Alaina Kronenberg
The term entoptic phenomena, derived from the Greek for “things perceived within vision,” refers to the visual sensations that arise from causes within the eye or visual system. In the most generally accepted context, the term describes the visual sensations produced or influenced by the native structures of one’s own eye or visual system that are not the customary result of forming an optical image of the external environment by the refracting system of the eye. These phenomena may result from either normal anatomic components of the eye or pathologic imperfections. Although these images are not the result of normal imaging in the external world, they may be perceived as if they are produced by objects in real space. However, special methods of illumination or other types of stimulation are required to adequately create and maintain these images. In addition, the direct attention and cooperation of the observer is a critical factor for the perception of these phenomena.
Many distinguished scientists (including Aristotle, Descartes, Helmholtz, and Edison) have contributed to our understanding of entoptic phenomena. Because of their depth and breadth, Purkinje’s studies are especially notable, and his name has become associated with many of the entoptic phenomena he first described.
Despite the fact that our understanding of the optical and physiologic bases of specific entoptic images remains inexact, these phenomena can be useful clinical tools. Entoptic phenomena have been used to study the pathophysiology of various ocular disorders, and they also provide a means to subjectively confirm objective findings. Entoptic imagery has been used to demonstrate the position of an opacity in the eye, measure the size of the foveal avascular zone, estimate leukocyte velocity, and the subjectively detect scotomas. However, it is important to always remember that subjective descriptions of these phenomena are notoriously inexact. Although a precise report of the visualization of a particular image can be useful, the inability to visualize an image is difficult to interpret and may simply reflect the patient’s failure to understand what they are being asked to observe or their inability to accurately describe what they do perceive.
In the text that follows, the topic of entoptic imagery is subdivided into a discussion of two broad categories. One category consists of effects that are primarily dependent on the optical properties of the preretinal ocular media. The second category includes phenomena that are principally dependent on the physiology of the retina and visual pathways. It should be recognized that this classification was adopted as an aid to organization and does not imply that the two categories are independent. On the contrary, visual sensitivity and neural processing necessarily affect the perception of entoptic images in the first category, whereas the visibility of many of the phenomena in the second category is reduced when the specific optical or illumination requirements are not met.
ENTOPTIC PHENOMENA ASSOCIATED WITH PROPERTIES OF THE OCULAR MEDIA
Various properties of the ocular media mediate the formation of entoptic images. The vergence of light within the eye together with the optical density and refractive nature of elements in the ocular media affect the appearance of entoptic images. The translucence of an element within the ocular media plays a major role in the type of image that is formed. Similarly, the location of an element relative to both the entrance pupil of the eye and the photosensitive surface of the retina have an important effect on the appearance of an entoptic image.
The position within the eye of elements producing entoptic images can be estimated by relative entoptic parallax.1 This principle originally was described by Listing as a useful technique for demonstrating the relative position of an opacity within the eye. Essentially, moving an illuminating source (usually a point source or pinhole) affects the perceived location of the entoptic images differentially, depending on their distance from the retina (Fig. 1). If the element is behind the entrance pupil, its movement appears to be opposite to the movement of the source. Conversely, if the element is in front of the entrance pupil, it appears to move in the same direction as the source. For example, lenticular or vitreous opacities show against movement while the eyelashes show with movement. The amount of parallactic movement depends on the distance from the entrance pupil; the movement appears greater the further anterior or posterior to the entrance pupil the element is located.
A more precise method for measuring relative entoptic parallax was developed by Brewster and modified by Donders (Brewster-Donders method for measuring the depth of opacity). In this method, a Scheiner disc with two small pinholes separated by approximately 2 mm. is positioned in the anterior focal plane of the eye. The field of view is then bounded by two overlapping circles whose width depends on the diameter of the exit pupil of the eye. Because the pinholes serve as two distinct sources, an opacity will cast two shadows on the retina. The distance between the two shadows, when projected onto a screen in real space, can be determined. As long as fixation is constant, this distance can be used to accurately estimate the location of the opacity.
HALOS
Because of irregular refractivity, translucent elements scatter transmitted light, reducing image contrast and forming halos. When the translucent element is more refractive than the surrounding ocular media, the entoptic image is relatively bright and surrounded by a dark rim (diffraction ring). A translucent element that is less refractive than its surrounding media produces a dark image surrounded by a light rim. If the translucent element is large or located close to the retina, a true scotoma results.
Ocular structures that act as diffraction gratings produce diffraction spectra when a point source is viewed. Entoptically, these diffraction spectra are visible as rainbow-like halos or coronas centered on the source. With a white light source, the outside rings of the diffraction spectra are red, while the inner rings are blue–violet. Halos are caused by diffraction from small regularly sized or spaced structures within the eye. The specific characteristics of individual halos depend on the structure of the diffraction grating underlying their generation. Diffraction theory predicts that the angular diameter of a halo is related directly to the wavelength of the light and inversely to the size (or in the case of a regular pattern to the spacing) of the diffracting structure. Therefore, as the diameter of the diffracting structures increases (e.g., cells, droplets) or the spacing of the elements in the diffraction grating gets larger (e.g., lens fibers) the diameter of the halo will become smaller. The longer the wavelength of light, the larger the diameter of the halo. The perceived diameter of an entoptic halo also depends on the distance between the diffracting structures and the retina. Halos are smaller when the diffracting structures are nearer to the retina.
Halos can result from either the normal physical properties of ocular structures or the alterations in these physical properties caused by the response of ocular tissues to pathology. In the healthy eye there are differences in size between halos associated with different ocular structures.2,3 With the exception of the zonular insertions and anterior cellular layer, the crystalline lens is composed of fibers that pass in an approximately radial manner from the anterior to the posterior sutures. As a result, the crystalline lens may be regarded as a strong positive lens with a radial diffraction grating (similar to any two- or three-dimensional array of lines or dots showing periodic variations of either transparency or refractive index) superimposed on the periphery. Because the axial portion of the lens appears to be relatively uniform, no lenticular halo is observed with a pupil less than 3 mm in diameter. With a dilated pupil, the effect of the peripheral lens becomes marked. In this case, the halo that generally is most visible results from diffraction by the radially arranged lens fibers. This produces a halo with diffraction rings that have been estimated to have diameters ranging from 4.5 degrees for violet, 5 degrees for blue, 5.4 degrees for green, and 6 degrees for yellow.4 A larger halo (approximately 9 degrees) was first described by Descartes in 1637 and appears to be caused by second-order diffraction by the corneal endothelium. A smaller halo (diameter of approximately 3 degrees) is a result of the structure and organization of the corneal epithelial cells, whereas the corneal endothelial cells and/or lens epithelial cells contribute to a slightly larger halo (approximately 4.5 degrees in diameter).
The halos associated with the response of ocular tissues to disease conditions include a halo of 7 degrees to 12 degrees that results from edema of the deeper layers of the corneal epithelium such as might be associated with elevated intraocular pressure (IOP), aphakic bullous keratopathy, and ultraviolet keratopathy. A larger (12 degrees to 14 degrees) halo is produced by debris such as mucus, blood, pus, or particulate matter on the corneal surface In some cases, the halo resulting from corneal edema can be differentiated from the lenticular halos by placing a stenopeic slit before the eye and restricting light entering the eye to a small zone of the entrance pupil (Fig. 2). Passage of light through a particular section of the lens generates a specific double section of lenticular halo. Moving a vertical stenopeic slit across the pupil gives moving sectors of light corresponding to the portions of the lenticular halo that are eclipsed (partly or wholly) according to the area of pupil that is occluded. Conversely, when the corneal stroma or epithelium becomes edematous, the regularity or spacing of the fibrils is disturbed, leading to small-body diffraction, with each element scattering light equally in all directions. As a result, the halo produced by corneal edema only appears reduced in intensity when a stenopeic slit passes in front of the eye.
SHADOWS
Nontranslucent structures absorb light and are opaque. Consequently, nontranslucent structures cast shadows. If the nontranslucent element is nearly the same size as the pupil or is situated close to the retina, these shadows are detectable. Otherwise, the shadow is not detectable because only the diffraction at the edges of nontranslucent elements produces light scatter. Therefore, the visual problems associated with nontranslucent elements are most severe when they are located posteriorly and produce a positive scotoma.
The pupil, lids, lashes, tear film, and corneal mucus can be observed entoptically as shadows. Under optimum conditions of illumination, the entoptic field is bounded by the shadow of the inner edge of the iris, which is visible as a bright patch limited by a circular ring with ragged edges corresponding to the pupillary margins. Irregularities of the pupillary margins as well as contraction and dilatation can be detected in this image, which also can be used to monitor the consensual pupil reaction driven by changes in illumination in the contralateral eye. Cogan5 used the entoptic visualization of the pupillary margins as the basis for a simple technique that permits patients to measure the size of their own pupil. When a pinhole is held close to the eye and a uniform field is viewed, the field appears to be the same size as the pupil. If two separate pinholes are viewed simultaneously, the patient sees two images the same size as the pupil. When the two spots appear juxtaposed, the distance between their centers equals the diameter of the pupil.
Superimposed on the bright image of the pupil, the shadows of the lashes appear to be jumping in and out of view when the lids are moved. In this image the lower lashes appear uppermost because of image inversion by the optics of the eye. The tear film and the mucus on the corneal surface also produce entoptic images. Horizontal striations that change with blinking typically result from visualization of the tear film. This can be demonstrated by partially closing and then opening the eyes while viewing a uniform field. A bright longitudinal stripe, with a dark margin caused by a ridge of tears, should be evident. Corneal mucus, as might result from inflammation, produces shadows that appear as bright spots surrounded by dark rings that move up and down as the palpebral fissure is narrowed or widened. Because mucus is very adherent to the cornea, these shadows can be quite persistent.
Folds in corneal epithelium create shadows that also appear as horizontal bands of light. These shadows are visible as unbroken lines extending across the entire width of the pupillary image, and they change their position as the eyelids are slowly opened or closed. Shallow linear channels in the corneal epithelium, such as those created by gentle digital pressure on the cornea through the closed eyelid or by rigid contact lens wear, also can be visualized entoptically. These shadows typically appear as mosaic patterns and are believed to be produced by elevated ridges in Bowman’s membrane that are created during the period of corneal flattening.
Structures in the lens and vitreous also cast shadows. The poor focusing of marginal rays through the pupil, caused by fibrous composition of the lens, produces images that appear as a system of rays that can be observed emanating from small bright objects on a dark background. A typical example is a star that appears to have a spiky surround. If the pupil is replaced by a smaller adjustable diaphragm, the rays (spikes) expand and contract with variations in the diaphragm. In cases in which the nucleus of the lens has a significantly different focus from the periphery, the central bright image may appear broken into multiple images (polyplopia). In addition, opacities in the lens and lenticular cataracts may be evident as dark, granular, stable patterns if their shadows extend to the retina. Alternatively, lenticular opacities may reduce retinal illumination and scatter light without producing detectable shadows.
Small inclusions in the vitreous (vitreous floater) may be visible entoptically as mobile shadows resembling bubbles, strings of pearls, or bundles of filaments. With a point source of white light, colored fringes may be detected bordering these shadows. Vitreous floaters can change shape when the eye moves. One form of vitreous floater casts a delicate, somewhat lacy or chain-like shadow that moves with gaze but tends to overshoot fixation and then return when the eye movement stops. Floaters in the anterior vitreous are perceived to move in a direction opposite to the movement of the eye, whereas floaters in the posterior vitreous move in the same direction as the eye movement. These entoptic visualizations often can be seen without the aid of a pinhole because they are caused by fine opacities located near the retina. On the basis of their appearance, these floaters have come to be known as muscae volitantes (Latin for flying gnats). Vitreous floaters are common, frequently visualized in the normal eye, tend to increase with increases in myopia or age and often are a source of concern to the patient. Once developed, a vitreous floater may persist for life, but the sudden onset of numerous floaters may be the initial sign of a retinal detachment. The vitreous floater associated with retinal detachment may represent blood particles in the vitreous. In cases in which these floaters disappear after a few days, it should not be assumed that the underlying problem has resolved.
ENTOPTIC PHENOMENA DEPENDENT ON THE STRUCTURE AND PHYSIOLOGIC PROPERTIES OF THE RETINA AND VISUAL PATHWAYS
IMAGES CAUSED BY THE RETINAL CIRCULATION
One of the most easily demonstrated of the entoptic visualizations is the subjective perception of the retinal blood vessels. Under ordinary circumstances, the shadows cast by the retinal vessels remain in fixed position relative to photoreceptors, even during eye movements. Because the underlying neural network is adapted, the vessels are not subjectively apparent in this situation. However, Purkinje noted that when light enters the eye from an unusual angle (e.g., obliquely through cornea or through the sclera) the shadows of the retinal vessels fall on unadapted retina and the shadows of the retinal vessels become visible for a brief period. This can occur, for example, during slit lamp biomicroscopy of the anterior segment. The shadows of the arterial and venous tree will be perceived as a greatly magnified, inverted image that has come to be known as either Purkinje’s tree or the Purkinje figure. In this image the retinal vessels are observed arising from the optic nerve head and branching out with finer and finer branches extending toward the fovea. With this type of viewing, the foveal pit, which lacks blood vessels, is readily distinguishable in this image as a vacant area surrounded by the branching pattern. Helmholtz made use of the parallax intrinsic to this phenomenon to demonstrate that the light-sensitive elements in the retina lay within the photoreceptor layer.
To visualize the retinal vessels, place a small, bright light source over closed eyelids near the limbal margins and oscillate the light source at a relatively low frequency (2 to 4 Hz). In the resulting entoptic image, the retinal vessels will appear as dark shadows against a yellow–orange background. To be able to perceive greater details in the image (such as the retinal capillaries), gaze at a uniformly illuminated, bright surface through a pinhole positioned at the anterior focal plane of the eye. The parallel light rays obtained with this technique enhance the contrast of the image so that shadows of vessels, including the capillaries surrounding the fovea, become visible. With sustained viewing, the Purkinje figure will fade, but the image can be regenerated by simply repositioning the illuminating source. By rotating the light source in a circular pattern, image stabilization can be avoided, and the shadows of the vessels will be perceived continuously.
Visualization of the retinal capillaries surrounding the fovea permits estimation of the size and shape of the foveal avascular zone. Applegate and colleagues6,7 used entoptic visualization of the retinal capillary net to measure the size and shape of the foveal avascular zone. They determined that the foveal avascular zone is an elliptical region with a mean diameter of approximately 0.755 mm and a major axis approximately 17% longer than the minor axis.7 These dimensions are consistent with foveal avascular zone diameters measured by angiographic techniques.8,9 Zeffren and co-workers10 also found that the point of fixation often is not centered in the foveal avascular zone. Furthermore, they observed that in a number of cases the point of fixation is located eccentric enough within the region that macular photocoagulation to within 200 μm of the center of the region could result in a lesion at the point of fixation, thereby compromising visual acuity.
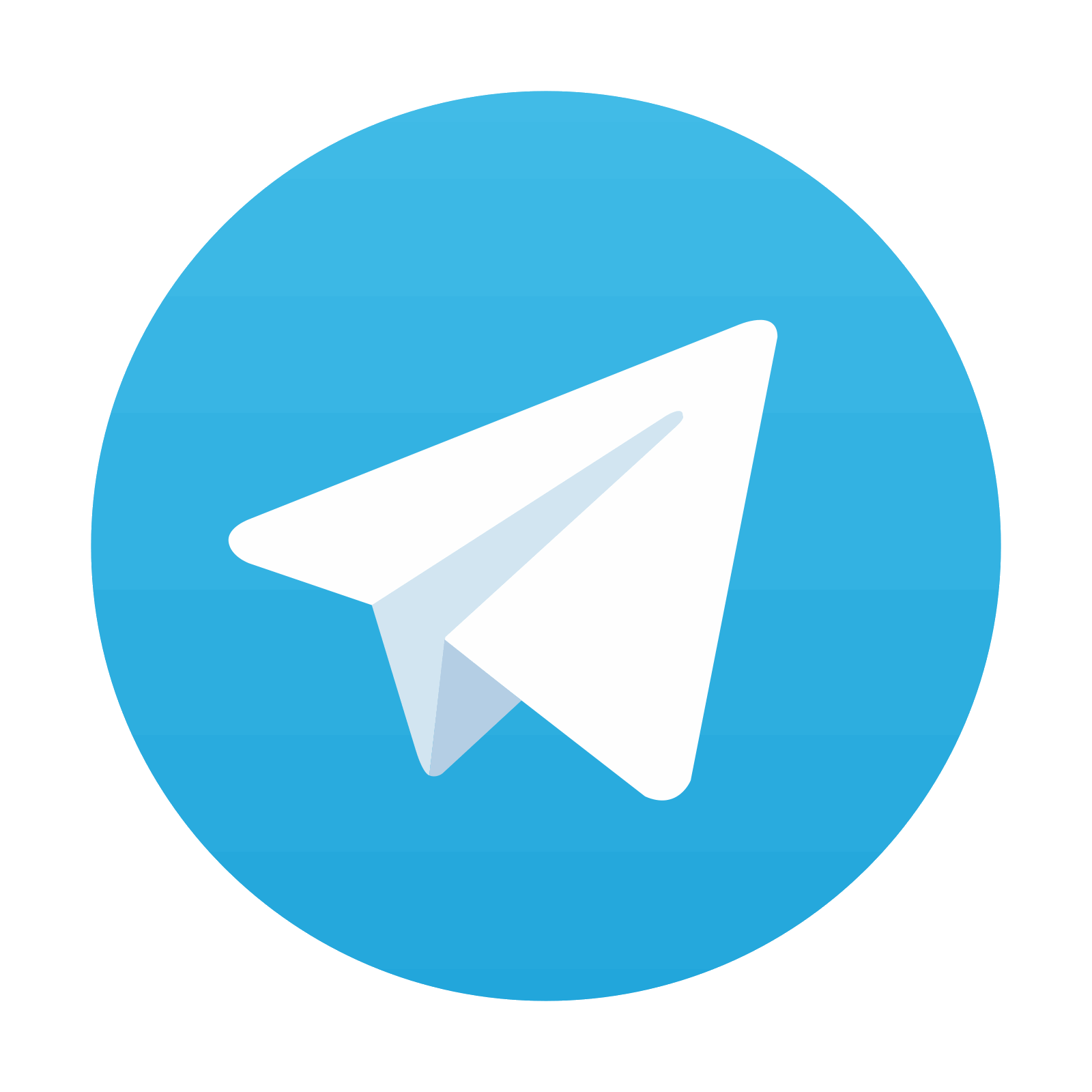
Stay updated, free articles. Join our Telegram channel
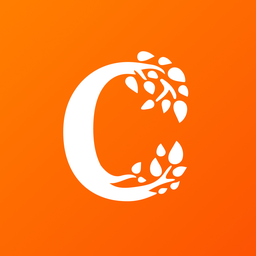
Full access? Get Clinical Tree
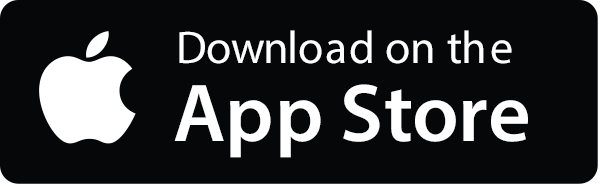
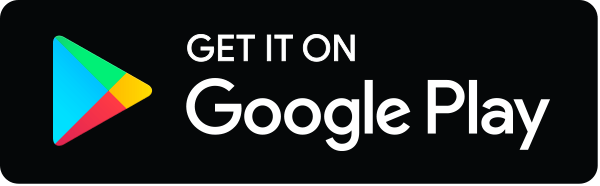