Embryology and Anatomy of Human Lenses
J. R. Kuszak
M. J. Costello
The cornea and the lens are the principal refractive elements of the eye. They are responsible for, respectively, stationary and variable refraction. However, although both must be transparent to function properly, the basis of their transparency is different. The cornea relies on the continuous pumping of interstitial fluid across its semipermeable surface membranes and a supramolecular organization of collagen fibrils for its clarity. Lens transparency is the result of a unique arrangement of its fiber-like cells, or fibers, and a gradient of refractive index produced by a variable crystallin protein concentration within these fibers. In fact, alterations in lens morphology affected during specific periods of development and growth, lead to quantifiable improvements in lens optical quality. Furthermore, lens structural anomalies affected during the same periods of development and growth, lead to quantifiable degradation in lens optical quality. Thus, the human lens is a prime example of form following function and malformation leading to malfunction.
In this chapter we describe lens anatomy throughout development, growth, and aging. In addition, we discuss how variations in lens structure influence its optics, physiology, and pathology.
LENS EMBRYOLOGY
PRIMARY FIBER FORMATION
Lens formation begins in the latter part of the first trimester as surface ectodermal cells, immediately overlying the developing optic vesicle, are induced to thicken and form the lens placode (Fig. 1). As the optic vesicle begins to fold inward and form the optic cup, the lens placode invaginates toward the developing optic cup until it eventually pinches off as an inverted (inside-out) lens vesicle. The apical surfaces of the lens vesicle cells are directed toward the lumen, whereas their basal surfaces are directed toward the outer surface. Throughout life, the basal surfaces of lens cells produce a progressively thicker basement membrane, the lens capsule, that envelops the lens. Lens development proceeds as cells of the lens vesicle approximating its retinal half are induced to terminally differentiate. A striking consequence of lens terminal differentiation is that the originally cuboidal lens vesicle cells are transformed into long fiber-like cells, or fibers. Fiber elongation proceeds until they fill in the lumen of the lens vesicle. Because these fibers are the first lens cells to be transformed into fibers, they are referred to as the primary fibers.
SECONDARY FIBER FORMATION
The cells of the lens vesicle that were not induced to form primary fibers remain as a monolayer, the lens epithelium, that covers the anterior surface of the primary fiber mass. Lens development and growth continues throughout life, in a manner similar to other stratified epithelia with the lens epithelium constituting the basal layer. However, whereas typically stratified epithelia have their progenitor cells distributed throughout the basal layer, the lens is unique in that its progenitor cells are sequestered as a distinct subpopulation within the lens epithelium known as the germinative zone (GZ). The remainder of the lens epithelial cells are sequestered in three additional regions known as the central zone (CZ), pregerminative zone (PGZ), and transitional zone (TZ). CZ epithelial cells comprise a broad polar cap of the lens epithelium covering most (approximately 80%) of the anterior surface of the lens. These cells are arrested in the G0 stage of the cell cycle and are not recruited to terminally differentiate into fibers.1 PGZ cells comprise a narrow, latitudinal band (approximately 5% of the lens epithelium) peripheral to the CZ. Although a small number of these cells undergo mitotic division, only rarely are any of their daughter cells induced to terminally differentiate and become additional fibers. Rather, these daughter cells add to the lens epithelial population as the anterior surface of the lens increases in size because of continued growth and aging.2,3 GZ cells comprise a narrow, latitudinal band (approximately 10% of the lens epithelium) peripheral to the PGZ. These cells undergo mitotic division, and some daughter cells are selected to terminally differentiate into additional fibers. Because these are the second fibers to develop, they are referred to as secondary fibers. Finally, TZ cells comprise a narrow, latitudinal band (approximately 5% of the lens epithelium) peripheral to the GZ. These cells are the nascent fibers having already begun the process of elongation.
FIBER ELONGATION
The secondary fibers comprise the layers, or strata, of the lens. An understanding of how fibers are added onto the existing lens throughout life is necessary to comprehend the complex structure of the lens that is paramount in the establishment and maintenance of its transparency (Fig. 2).
As the cells within the TZ migrate posteriorly, they rotate 90 degrees about their polar axis while elongating bidirectionally. As fiber elongation continues, the anterior ends are insinuated between the lens epithelium and the primary fiber mass while the posterior ends are insinuated between the primary fiber mass and the posterior lens capsule. Elongation is complete when the anterior and posterior ends of newly formed secondary fibers break contact with the apical surfaces of CZ epithelial cells anteriorly and the capsule posteriorly, respectively. The fact that all fibers detach from the basement membrane, the lens capsule, is direct evidence that the lens is a stratified, rather than a simple epithelium because in simple epithelia, all cells permanently retain contact with the basement membrane.
The anterior ends of fully elongated fibers abut and overlap with one another, as do the posterior ends, to form a growth shell. Because secondary fiber formation, or stratification, occurs throughout life, the net result is the establishment of successive growth shells, surrounding the original primary fiber mass. Thus, a view through a lens split along its anteroposterior or visual axis reveals concentric growth shells and/or radial cell columns (Fig. 3). The inside-out development scheme of the lens dictates that growth shells become progressively more internalized and, therefore, fibers cannot be sloughed off. In fact, all primary and secondary fibers formed are retained and must be supported for a lifetime. Failure to preserve the viability of any fiber is presumed to lead to pathology.
After fiber elongation is complete, terminal differentiation continues as the newly formed fibers routinely eliminate their nuclei, Golgi bodies, rough endoplasmic reticulum, and most smooth endoplasmic reticulum and mitochondria.4,5,6,7,8,9,10,11 The removal of these organelles is often described as necessary because their retention would cause a significant diffraction of light and thereby compromise lens function. However, it should be noted that other stratified epithelia (e.g., skin) also routinely eliminate these same organelles from cells in upper or older layers as a function of terminal differentiation. Fiber maturation continues with the production of specialized cytoplasmic proteins, the crystallins, as well as specialized cytoskeletal and plasma membrane components. As fibers age, the structural and biochemical nature of the previously described fiber characteristics are altered.12
LENS SUTURES
A common misconception is that all fully elongated fibers are meridians, crescent-shaped, fusiform cells that are widest at their midportion and tapered to a point at their ends. A logical extension of this misconception is that the ends of all secondary fibers in any growth shell extend to confluence at the anterior and posterior lens poles. Although such a description of gross fiber shape, length, and end-to-end organization is fairly accurate for some vertebrate lenses, most notably avian, it grossly oversimplifies the intricate variations in fiber shape and length that result in the highly ordered arrangement necessary for a human lens to function properly.
Human fibers are not meridians. They neither extend from pole to pole nor have tapered ends. In fact, human fibers are more than three times as wide, or flared at their ends, than at their midportions. Furthermore, the entire length of most fibers does not lay within a plane coincident with the visual axis as defined by its equatorial location. Instead, most fibers have two types of curvature: a convex-concave curvature that conveys the fiber’s crescent appearance and end curvature, the curvature of anterior and posterior end segments of fibers, within any growth shell in opposite directions away from the polar axis. Together, these two curvatures convey on fibers a three-dimensional (3D) S shape (see Fig. 2).
Y SUTURE OF THE FETAL NUCLEUS
The ends of fibers abut and overlap within and between growth shells to form distinct latitudinal arc segments. Individually these arc segments are referred to as suture branches, and collectively they make up defined “suture patterns” within each growth shell. Fiber shape, end taper or flare, and the extent of end curvature combine to determine the number of suture branches and resulting suture patterns (Fig. 4).
Growth shells are composed of two types of secondary fibers: straight and S-shaped fibers. A straight fiber is crescent-shaped, with its entire length lying within a plane passing through the visual axis as defined by its equatorial location (Fig. 5, upper left). However, only one end of a straight fiber extends to a pole. Throughout embryonic development, each growth shell normally contains six straight fibers. In the anatomic position, these straight fibers are normally positioned equidistantly around the lens equator beginning with the most superior equatorial location. Thus, each growth shell is effectively subdivided into sextets.
In addition to their crescent shape, S-shaped fibers have anterior and posterior end segments that exhibit precise curvature away from the poles inopposite directions (see Fig. 5, upper right). Neither of the ends of an S fiber extends to a pole nor does its entire length lie within a plane passed through the visual axis as defined by its equatorial location. S fibers are arranged as distinct groups, positioned between straight fibers. Because of opposite-end curvature, within any embryonic growth shell, the anterior and posterior ends of S fibers become aligned as offset anterior and posterior latitudinal arc lengths (see Fig. 5, middle left). The anterior ends of proximal groups of S fibers abut and overlap within and between successive growth shells to produce anterior suture branches (see Fig. 5, middle right). However, although the anterior ends of fibers in proximal groups form anterior suture branches, opposite-end curvature dictates that their posterior ends form precisely offset posterior branches with different groups (see Fig. 5, lower left). The origin of anterior suture branches is defined by the ends of straight fibers that extend to confluence at the posterior pole but not to the anterior pole. Similarly, the origin of posterior suture branches is defined by the ends of straight fibers that extend to confluence at the anterior pole but not to the posterior pole. All suture branches extend to confluence at the poles and combine to form discrete anterior and posterior suture patterns. Throughout development the three anterior suture branches are normally oriented at 120 longitudinal degrees to one another to form a Y suture pattern (see Fig. 5, lower right). It follows then that the three posterior suture branches are also normally oriented at 120 degrees to one another but because of opposite-end curvature are offset 60 degrees to the anterior suture branches to form an inverted Y suture pattern.
As additional growth shells are added throughout fetal development, each growth shell normally consists of radially longer and identically shaped secondary fibers (Fig. 6, upper left). Thus, as the Y suture branches of each growth shell become overlain in successive shells, the anterior and posterior ends of all fibers become arranged as right triangle-shaped suture planes extending from the primary fiber mass to the lens periphery (see Fig. 6, upper right, middle, and lower rows). The three anterior suture planes are normally oriented at 120 degrees to one another as are the three posterior suture planes. However, as a result of opposite-end curvature, all six of the Y suture planes are normally offset by 60 degrees to one another.
STAR SUTURES OF THE JUVENILE AND ADULT NUCLEI AND CORTEX
After birth and for the rest of life, primate lenses normally form progressively more complex “star suture” patterns during infancy, adolescence, and adulthood. The evolution of the “simple star suture,” produced during infancy, is depicted in Figure 7. As additional growth shells are added after birth, specific S-shaped fibers are overlain by a pair of new straight fibers. Because the ends of straight fibers delimit suture branches, new groups of S-shaped fibers with less end curvature are defined on either side of the new straight fibers. The ends of these fibers abut and overlap to form two new evolving suture branches that extend to confluence at one of the original three Y suture branches (see Fig. 7, upper middle). At this point S-shaped fibers are arranged in eight unequal groups positioned between eight straight fibers.
As more growth shells are added (see Fig. 7, upper right), the new pair of straight fibers are overlain by longer straight fibers, and a second new pair of straight fibers are also added. The S-shaped fiber groups on either side of the original new pair of straight fibers are longer, forming two longer evolving suture branches that extend to confluence farther along an original Y suture branch. At the same time the ends of the second new pair of straight fibers delimit additional new evolving suture branches that extend to confluence at a second of the original three Y suture branches. At this point, the S-shaped fibers are arranged into 10 unequal groups positioned between 10 straight fibers.
As more growth shells are added (see Fig. 7, lower left), the first new pair of straight fibers are overlain by even longer straight fibers, the second new pair of straight fibers are overlain by longer straight fibers, and a third new pair of straight fibers are added. The ends of the S-shaped fibers positioned on either side of the first pair of straight fibers now form two complete new suture branches that extend to confluence at the poles. At the same time, the ends of S-shaped fiber positioned on either side of the second pair of new straight fibers continue forming evolving suture branches that extend to confluence farther along the second of the original three Y suture branches. The ends of the third new pair of straight fibers delimit additional new evolving suture branches that extend to confluence at the third of the original three Y suture branches. At this point, S-shaped fibers are arranged into 12 unequal groups positioned between 12 straight fibers.
As more growth shells are added (see Fig. 7, lower middle), the second new pair of straight fibers are overlain by longer straight fibers, and the third new pair of straight fibers are overlain by longer straight fibers. The groups of S-shaped fibers positioned on either side of the second new pair of straight fibers, now form two additional new suture branches that extend to confluence at the poles. At the same time, the ends of the third pair of new straight fibers form longer evolving suture branches that extend to confluence farther along the third of the original three Y suture branches. As the final infantile growth shells are added (see Fig. 7, lower right), all three new pairs of straight fibers continue to be overlain by straight fibers. All the groups of S-shaped fibers positioned on either side of all the new pairs of straight fibers form a symmetrical, six-branch simple star anterior and posterior suture that are offset by 30 degrees.
As a result of forming a simple star suture over the period of infantile development, radial cell columns now contain fibers that have neither identical shape nor lengths that are a simple radial function (Fig. 8, first row). The ends of fibers, and by extrapolation suture branches, are out of register in successive growth shells. Thus, discontinuous suture planes are formed from the fetal nucleus to the lens periphery (see Fig. 8, second row).
Throughout adolescence and adulthood, star (see Fig. 8, third row and Fig. 9) and complex star sutures (see Fig. 8, fourth row), respectively, the third and fourth generations of sutures in primate lenses, are formed in a similar manner. The fully formed anterior and posterior star sutures, offset by 20 degrees because of opposite-end curvature, are normally characterized by 18 groups of S-shaped fibers, with even less opposite-end curvature, positioned on either side of 18 straight fibers. Finally, the fully formed anterior and posterior complex star sutures, only offset by 15 degrees because of the least amount of opposite-end curvature, are normally characterized by 24 groups of S-shaped fibers positioned on either side of 24 straight fibers.
![]() Fig. 9. Low magnification scanning electron microscopy montage of a young adult human lens star suture (see Fig. 8). |
ZONES OF DISCONTINUITY
The zones of discontinuity, seen in human lenses with transillumination slit-lamp biomicroscopy, contain the four progressively more complex generations of sutures. Thus, abnormal slit-lamp profiles of cataractous lenses (e.g., diabetic and cortical) contain a record of abnormal suture development.13 Slit-lamp biomicroscopy also reveals a thin equatorial band, or region of minimal light scatter, commonly referred to as the central sulcus. The thickness of the central sulcus is the thickness of the embryonic nucleus. Thus, it only includes embryonic nuclear fibers and portions of the midsegments of fetal, infantile, juvenile, and adult nuclear fibers, all aligned parallel to the anteroposterior axis. As aresult, the central sulcus is characterized by a complete lack of sutures within its boundaries.
SUTURES AND LENS OPTICAL QUALITY
The functional significance of increasingly complex suture patterns formed throughout life is as follows: As light rays pass through uniformly shaped fibers arranged in radial cell columns, they repeatedly encounter fiber membrane, cytoplasm filled with crystallin proteins, fiber membrane, extracellular space, and so forth in relatively constant increments. Cumulatively, the lens is transformed into a series of coaxial refractive surfaces or into a structure that minimizes light diffraction.14 This theory of lens function has been proven to be accurate by correlating lens sharpness of focus, ascertained by low power helium neon laser scan analysis, with lens structure, as assessed by light (LM) and electron microscopic (EM) analysis.15,16,17,18 However, suture branches are formed by the overlap of irregularly shaped fiber ends. In the Y suture, formed before birth, branches are overlain in successive growth shells to form continuous, irregular suture planes extending from the embryonic nucleus to the lens periphery. Thus, light rays passing through these planes would repeatedly encounter fiber membrane, cytoplasm with crystallins, fiber membrane, extracellular space, and so forth in increments that are not constant, causing a reduction in lens sharpness of focus. However, as lenses grow and age, branches are not overlain in successive growth shells, and, thus, they form discontinuous suture planes extending from the fetal nucleus to the lens periphery. Then as light rays pass through the lens, they encounter fewer instances of constant increments of fiber membrane, cytoplasm with crystallins, fiber membrane, extracellular space, and so forth characteristic of nonprimate lens suture planes. This explains in part why primate lenses are optically superior to nonprimate lenses.
SUTURES AND LENS PHYSIOLOGY
Lenses lack a blood supply. Nourishment derived from the aqueous and vitreous humors enters the lens mass through simple diffusion. However, the effective depth for nutrient diffusion is restricted to the outer 10% of the lens radius.19 Thus, only the lens epithelium and the peripheral superficial cortical fibers, which have full complements of organelles, have effective access to critical nutrients. In contrast, the cortical and nuclear fibers, which lack organelles, have limited access to nutrients, and this nutrient delivery problem is amplified as the lens mass grows throughout life. Lens sutures may play an important role in supporting an internal circulatory system in the lens.
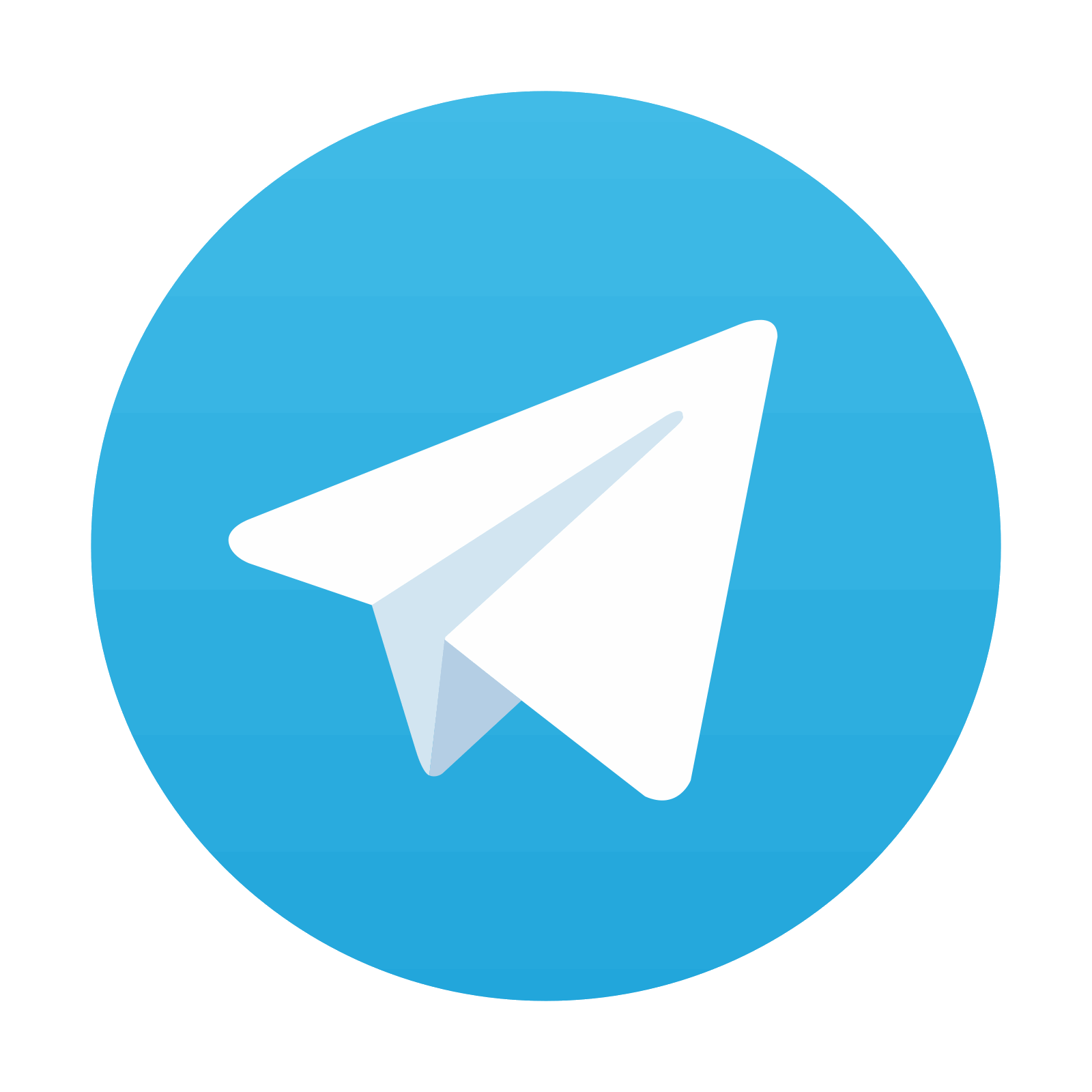
Stay updated, free articles. Join our Telegram channel
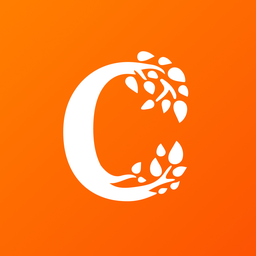
Full access? Get Clinical Tree
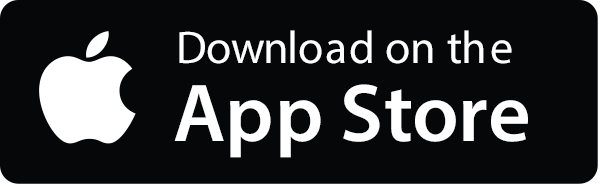
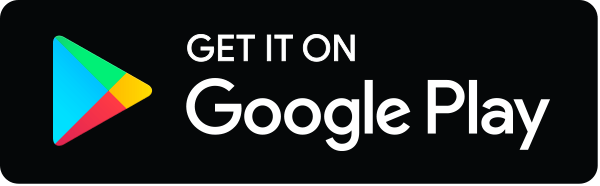