Artificial Vision, Retinal Implants, and Implantable Telescope
Nancy Kunjukunju
Vision results from light transmission. Light is converted to electrical impulses that are then relayed to the brain. After traveling through the cornea and lens, light reaches the retina and the optic nerve and is converted to electrical energy. This energy is conducted intracranially through the lateral geniculate nucleus to the primary visual cortex in the occipital lobe. The information is subsequently transmitted to higher cortical areas of the brain, thus making the optic nerve and retina vital components of the pathway of light transmission.
According to a recent report by the World Health Organization, 285 million people are visually impaired worldwide, 39 million are blind, and 246 million have low vision (October 2011 Fact sheet WHO). Blindness, the inevitable result of irreparable damage to the visual pathway, is often a result of injury to either the neural retina or the optic nerve. Retinitis pigmentosa (RP) is a leading cause of inherited visual blindness, while age-related macular degeneration (AMD) is a leading cause of degenerative visual loss in the elderly.
Research is currently under way to offer patients suffering from visually debilitating diseases viable prosthetic options for visual rehabilitation. Approaches to visual rehabilitation involve electrical microstimulation of the visual cortex, the lateral geniculate nucleus, the optic nerve, and the retina to generate the impression of light or phosphenes. Beginning in the early 1900s, experiments in cortical stimulation were performed in attempt to assist the blind. For example, in 1929, the German neurosurgeon Foerster observed that electrical stimulation of the cortex enabled his subject to detect a spot of light. This phenomenon of electrically induced light perception was defined as a phosphene (1).
In 1974, an electrode was used in a blind patient to stimulate the visual cortex to generate light perception (2). Experiments conducted by Dobelle et al. and Normann et al. (3,4,5,6) utilized either implanted surface electrodes or intracortical microelectrodes to excite the visual cortex. Over 50 electrodes were implanted over the occipital pole, and although some form of visual perception was obtained in the experiment, it was difficult to control the number of phosphenes induced by each electrode and the interaction between phosphenes. Furthermore, electrical stimulation was seen to cause an increase in the incidence of epileptic activity and to cause meningeal pain induced by high currents and large electrodes.
A contemporary form of the intracortical prosthesis, the Utah electrode array, consists of multiple silicon spikes. In testing of the array, the silicon tissue reaction ranges from none to gliosis and buildup of fibrotic tissue between the array and meninges (7). Recently, research was conducted to determine the feasibility of implanting a neural prosthesis in the lateral geniculate nucleus of the thalamus, which relays visual information from the retina to the visual cortex (8). Initial research demonstrated that electrical stimulation of the lateral geniculate body can generate neural responses in the visual pathway: electrical stimuli delivered
to the thalamus engendered responses similar to those of when images are presented to the eye. Further research is currently under way.
to the thalamus engendered responses similar to those of when images are presented to the eye. Further research is currently under way.
Cortical prosthesis may bypass diseased visual pathway neurons rostral to the primary cortex and allow for visual restoration regardless of the insult to the eye. However, the organization of the visual field is more complex at the level of the primary cortex than at the level of the optic nerve or retina and may result in limited spatial resolution. Intracranial surgery also runs the risk of central nervous system (CNS) infection, epilepsy, and disturbance of blood flow to the optic nerve. Surgical complications carry significant morbidity and mortality risks for the patient.
Given the potential dangers of approaching artificial vision from the cortical approach, other groups have chosen to pursue an ocular prosthesis from within an eye possessing remaining viable tissue. Previous reports show that in spite of the level of vision loss in patients with RP, viable cells in the eye are able to transmit information with 30% of ganglion cells and 80% of inner nuclear layer cells that remain histologically intact after the death of photoreceptors (9). Investigations are ongoing in regard to a viable ocular prosthesis at the levels of suprachoroidal, subretinal, and epiretinal space by utilizing the preexisting signal processing network. This requires that sufficient bipolar cells or ganglion cells remain to elicit a response to electrical stimulation. In cortical prosthesis experiments, an array of electrodes is used to deliver an electrical current to the retina, thus stimulating functional retinal neurons to send signals to the visual cortex to be perceived as phosphenes (Fig. 26.1). In contrast to cortical electrodes, the use of preexisting signal processing network is expected to have higher visual resolution.
![]() Figure 26.1 ▪ Light is transmitted through the anterior segment of the eye to reach the posterior elements and reaches the electrodes at either the subretinal or epiretinal layer. |
Sakaguchi and Tano et al. (10) investigated suprachoroidal transretinal stimulation in which an electrode was inserted into the suprachoroidal space to elicit electrical evoked potential (EEP). In the subretinal approach, explored by Chow et al. (11,12,13) and Zrenner et al. (14), lost photoreceptor function was replaced by a subretinal microphotodiode array (MPDA) that activated the remaining functioning retinal network. In the epiretinal approach, investigated by Eckmiller et al. (15), Humayun et al. (16,17), Rizzo et al. (18,19), and Walter et al. (20,21), devices stimulated the ganglion cells from the vitreous side of the retina.
SUPRACHOROIDAL-TRANSRETINAL STIMULATION
A new approach in artificial retina stimulation considers suprachoroidal-transretinal stimulation (STS) to elicit phosphenes. In 2010, STS implantation was shown to be safe and functional in two patients with advanced RP (22). This method involves insertion of a retinal prosthesis in the sclera pocket and placement of a reference electrode in the vitreous cavity. The device consists of a secondary coil that receives signals from an external coil and a decoder that generates pulses, which are then delivered to individual electrodes. The internal device was implanted under the skin of the temporal portion of the skull, and a 49-electrode array was implanted in the sclera pocket of one eye (Figs. 26.2 and 26.3).
Functional testing using head scanning revealed that the detection and discrimination of objects was possible with a small number of active electrodes. The resolution of the image with STS prosthesis may be lower because the electrodes
are farther from the retina than they would be when utilizing either the subretinal or epiretinal approach. In addition, there is a potential need for greater stimulation thresholds, and a demand for increased power given the added distance. It is considered safer, however, as the electrodes do not touch the retina and are stably fixed in the sclera pocket.
are farther from the retina than they would be when utilizing either the subretinal or epiretinal approach. In addition, there is a potential need for greater stimulation thresholds, and a demand for increased power given the added distance. It is considered safer, however, as the electrodes do not touch the retina and are stably fixed in the sclera pocket.
![]() Figure 26.2 ▪ Diagram of retinal prosthesis system. A. Lateral view of the skull XP of Pt 1 after implantation surgery. A, Position of skin incision to insert and anchor the device. B, Position of skin incision to fix the cable to the bone of the lateral orbital wall. C, Return electrode. D, Stimulating electrode. E, Decoder. F, Secondary coil. B. The implanted devices, cable, and electrodes. Scale bar, 3 cm. (Reprinted with permission from Fujikado T, Kamei M, Sakaguchi H. Testing of semichronically implanted retinal prosthesis by suprachoroidal-transretinal stimulation in patients with retinitis pigmentosa. Invest Ophthalmol Vis Sci. 2011;52(7):4726. Copyright 2011. The Association for Research in Vision and Ophthalmology, Inc.) |
SUBRETINAL IMPLANTS
In 1956, a retinal prosthesis was described in which a lightsensitive selenium cell was placed behind the retina of a blind patient, allowing the patient to discern a moment of light perception (23). Subsequently, microchips containing light-sensitive MPDAs were implanted between the neural retina and the retinal pigment epithelium. Access to the subretinal space is gained through internal or external mechanisms. It is achieved externally by a process in which the sclera is dissected down to the choroid, the choroid is excised, and the implant is placed in the desired location under the retina. Internal access is accomplished by a vitrectomy and retinotomy (24). A combination of the two methods may also be employed: fluid is used to detach the retina internally, and a choroidal incision is made externally. MPDAs are used to replace degenerated photoreceptors by rendering the transmitted light into small currents proportional to the light stimulus. Essentially, there is direct replacement of lost photoreceptor function.
In the case of hereditary retinal degenerations, the remaining intact neural tissue may be used to transmit and process image information. Subretinal implants require less current for stimulation because they are placed anatomically closer to the surviving bipolar cells, and they do not need adhesives or mechanical fixation. Inserting the microchip under transparent retina allows the microchip to sense light while simultaneously generating a signal through use of an array. Additionally, there is no need for either external cameras or processing units. It was considered that subretinal implantation may affect neurotrophic factors and enable a level of neuroprotection to the remaining viable tissue. Electroretinograms were assessed, which led to later suggestions that subretinal stimulation may preserve photoreceptors (25).
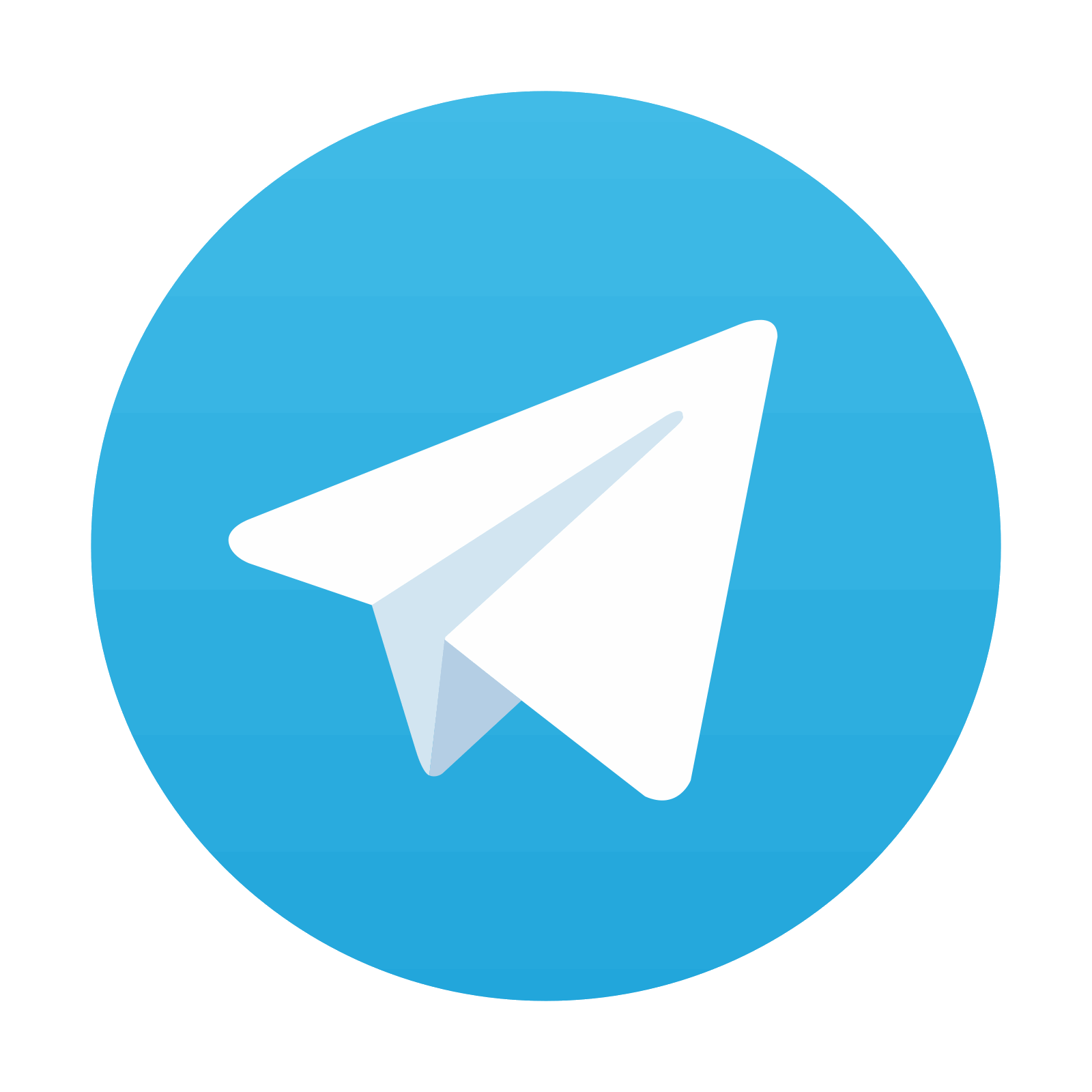
Stay updated, free articles. Join our Telegram channel
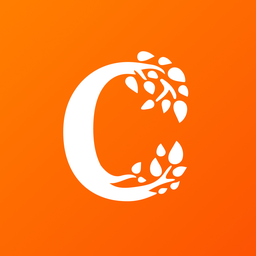
Full access? Get Clinical Tree
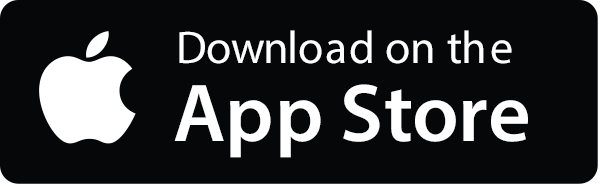
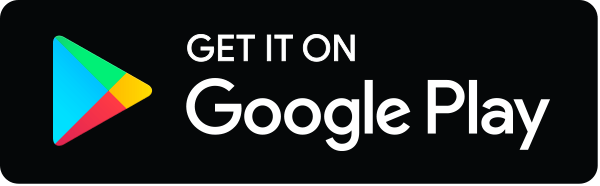