Aqueous Humor: Secretion and Dynamics
B’Ann True Gabelt
Julie A. Kiland
Baohe Tian
Paul L. Kaufman
The aqueous humor is a transparent, colorless solution continuously formed from plasma by the epithelial cells of the ciliary processes. It is secreted into the posterior chamber, passes from the posterior chamber through the pupil into the anterior chamber, and is drained at the anterior chamber angle. Most of the aqueous drains into the venous circulation via the trabecular meshwork, Schlemm’s canal, scleral collector channels, and aqueous and episcleral veins; the remainder drains into the orbit via the interstices of the ciliary muscle, the suprachoroidal space, and the sclera (Fig. 1). The composition and formation of aqueous resembles that of cerebrospinal fluid.1 Aqueous humor is thought to serve several functions:
Aqueous delivers oxygen and nutrients to, and removes waste products, blood, macrophages, inflammatory products, or other debris from the posterior cornea, crystalline lens, and perhaps the anterior vitreous, structures that are necessarily avascular.
Continuous formation and drainage of the aqueous helps maintain the intraocular pressure (IOP), necessary for maintaining the shape and internal alignment of the ocular structures and, consequently, optimal optical properties.
The aqueous maintains a transparent and colorless medium of lower refractive index between the posterior cornea and the lens, and thus constitutes an important component of the eye’s optical system.
Circulation of the aqueous in the anterior chamber occurs via hydrostatic phenomena, including mechanical forces caused by eyeball and head movements, thermal currents resulting from the temperature differential between the warmer vascular iris and the cooler avascular cornea, and the pressure gradients between the posterior chamber, anterior chamber, and episcleral veins. As the fluid bathes the anterior lens, iris, and corneal endothelium, its composition is altered as a result of the exchange of nutrients, cellular waste products, and other substances within these structures. The entire volume of the aqueous humor is replaced every 90 to 100 minutes.2
Continuous formation and drainage of the aqueous is essential to the good health of the eye. In the absence of aqueous circulation, the cornea is thickened, the anterior chamber is absent, the iris is partly atrophic, and the lens is cataractous.3
This chapter reviews the anatomy and physiology of aqueous humor circulation from formation to drainage. The ciliary body and its secretory mechanisms, the blood–aqueous barrier, the aqueous humor composition, the methods of measuring the aqueous flow rate and factors affecting it, the pathways of aqueous flow within the eye, and the aqueous outflow system are all discussed.
ANATOMY OF THE CILIARY BODY
The ciliary body forms a ring along the inner wall of the eyeball, and extends anteriorly from the scleral spur and iris to the ora serrata posteriorly. The greater part of the ciliary body mass is accounted for by the ciliary muscle, the bundles of which are arranged in three regional orientations: radial, circular, and longitudinal. When viewed in transverse section, the ciliary body appears as an isosceles triangle (Fig. 2). The base of the triangle faces anteriorly, while one of its sides lies along the sclera, separated from it only by a potential space continuous with the suprachoroidal space. The other side, or inner portion of the ciliary body, is divided anatomically into two parts: the posterior portion (pars plana) and the anterior portion (pars plicata). Projecting inwardly from the pars plicata are approximately 70 villus-like structures: the ciliary processes. Viewed posteriorly, the ciliary processes appear as radial ridges, to which collectively the name corona ciliaris has been given. It is the ciliary processes that are responsible for aqueous formation.
The ciliary processes are long and slender in early life but become more blunt in later years. Their dimensions vary but average 2 mm in length anteroposteriorly, 0.5 mm in width, and 1 mm in height.4 The processes are greatly convoluted. Structurally, they consist of capillaries surrounded by a loose connective tissue, encircled by a double epithelial layer. This structure provides a large surface area (in rabbits5 approximately 5.7 cm2) for capillaries to be in close proximity to the double layer of epithelium and for the epithelium to face the posterior chamber. This arrangement maximizes access of the ciliary body secretions into the small space of the posterior chamber.
The blood supply of the ciliary body has a dual origin.4,6,7,8,9,10,11,12,13 The two (medial and lateral) long posterior ciliary arteries, which penetrate the sclera posteriorly and travel anteriorly in the suprachoroid, give rise to the major arterial circle of the iris, the vascular structure that supplies the inner and anterior division of the ciliary muscle, ciliary processes and iris.13 The seven anterior ciliary arteries, which penetrate the sclera anteriorly after supplying the extraocular rectus muscles, also contribute to the major iris arterial circle, and to the outer and posterior areas of the ciliary muscle, and the peripheral (anterior) area of the choroid. The microvasculature of the ciliary processes themselves, arising from the short radial ciliary arteries, which in turn arise from the major arterial circle of the iris, is arranged into three distinct vascular areas. The first of these is located at the anterior end of the major processes, with venous drainage achieved via venules passing the ciliary body. The second and third vascular areas supply the major and minor ciliary processes, and are drained posteriorly by venules located at the margin of the ciliary processes. The system of ciliary process venules in turn drains mainly through the vortex system of the choroid. Each short radial ciliary artery has many branches, providing an extensive capillary network (Fig. 3). The capillaries of the ciliary processes are large, thin-walled, and highly fenestrated. Thus, the capillary network of the ciliary processes provides a large surface area of highly permeable vessels to initiate the process of aqueous formation.
The capillaries are surrounded by the stroma, which consists mainly of loose connective tissue and collagen. A basement membrane, which is the thickened anterior continuation of Bruch’s membrane separating choroid and retinal pigment epithelium, separates the stroma from the epithelial layers.
The ciliary epithelial cells have been subject to intensive study by light and electron microscopy. A striking feature is the interdigitation of the lateral surfaces of adjacent cells and the basal infoldings (Fig. 4), which are characteristic features of secretory epithelia concerned with fluid transport.14 The relation of the two epithelial cell layers is of importance because as the secreted aqueous is derived from an ultrafiltrate of blood in the stroma of the ciliary body, transport must occur across both layers. The double-layered ciliary epithelium itself is derived from anterior continuations of the retinal pigmented epithelium (forming the pigmented layer), and the neuroepithelium from which the retinal cells are derived (forming the nonpigmented layer). However, during embryogenesis, invagination of the neuroepithelium occurs, with the result that the apical surfaces of each cell layer in the ciliary epithelium face one another, while the basolateral surface of the nonpigmented layer faces directly into the posterior aqueous chamber. Conversely, the basolateral surface of the pigmented layer is tightly bound to the basement membrane. Cells of the pigmented epithelium contain many melanin granules. Gap junctions are present between the lateral interdigitations of the pigmented cells. Desmosomes also occur between the lateral interdigitations of the pigmented and nonpigmented epithelia and between their apical membranes.
The cells of the inner nonpigmented epithelium possess numerous intermediate-sized mitochondria, and the rough endoplasmic reticulum is particularly well developed, indicative of active protein synthesis. Small vacuoles may be present in large numbers near the apex of these cells. Occasionally, a few pigmented granules may be seen. Tight junctions are present in the lateral interdigitations between the nonpigmented cells (Fig. 4), thus forming a barrier for the passage of larger molecules between the cells. This important physiologic barrier, constituting part of the blood-aqueous barrier, is discussed further in a later section.
The internal limiting membrane of the ciliary body is a more complex and thicker structure than its posterior counterpart on the retina, in part because it serves as the basement membrane for the inverted nonpigmented ciliary epithelium, and as the site of insertion of the zonular fibers,4 to which the lens is attached.
FORMATION AND SECRETION OF AQUEOUS HUMOR
Early in the twentieth century, aqueous humor was regarded as a stagnant fluid.15 However, this misconception was revoked after a number of experiments designed to investigate this were carried out, including Seidel’s procedure,16 in which a cannula connected to a reservoir of indigo carmine dye was inserted into the anterior chamber of the rabbit eye. The reservoir was raised, thus creating a pressure of 15 mm Hg, and the dye was seen to enter the anterior chamber and subsequently the episcleral veins. From this, it was concluded that aqueous humor is continuously formed and drained, and it is to a large extent from this historic work that the modern study of aqueous humor dynamics has developed.
Other aspects of the anatomy and physiology of aqueous drainage were discovered subsequently. Boerhaave first described the presence of the aqueous veins,17 and Ascher18 observed a clear fluid in veins of the episclera and demonstrated by means of external compression with a glass rod that these veins were interconnected with veins containing blood. Goldmann19 demonstrated that these vessels contained aqueous humor by injecting fluorescein intravenously and observing the dye entering the anterior chamber and subsequently the aqueous veins. Ashton20 identified an aqueous vein in a living human eye, and postmortem examination using a neoprene cast showed that there was a direct passage between the vessel and Schlemm’s canal.
Three physiologic processes are known to contribute to the formation and chemical composition of the aqueous. These are diffusion, ultrafiltration (and the related dialysis), and active secretion. The first two are passive and, therefore, require no active cellular participation. Diffusion of solutes across cell membranes occurs down a concentration gradient. Substances with high lipid solubility coefficients that can easily penetrate biological membranes move readily in this way. Ultrafiltration is the term used to describe the bulk flow of blood plasma across the fenestrated ciliary capillary endothelia, into the ciliary stroma, which can be increased by augmentation of the hydrostatic driving force. This process is responsible for the formation of the reservoir of the plasma ultrafiltrate in the stroma, from which the posterior chamber aqueous is derived, via active secretion across the ciliary epithelium. Active secretion requires energy, normally provided via the hydrolysis of adenosine triphosphate (ATP). The energy is used to secrete substances against a concentration gradient.
DIFFUSION
Diffusion arises from the fact that the molecules in a fluid are in constant, random motion. The magnitude and rate of motion vary directly with the temperature. If the molecules in a liquid or gas are not evenly distributed, then simply by the laws of statistical probability the molecules will eventually reach a state of equilibrium whereby they are redistributed equally. For example, if there is initially a cloud of smoke particles on the right side of a closed room without air currents, more particles will move from the right side to the left, than from left to right. This process will continue until there is a relatively even distribution throughout the room, at which time the number of particles going from right to left at any moment will be equal to the number moving in the opposite direction.
A similar process occurs in single solutions and in situations in which two solutions are separated by a membrane, as long as the membrane is permeable to at least some of the constituents of the solution (semipermeable membrane). Most capillary walls are permeable to water, dissolved gases, and many small molecules and ions. In a stagnant system, substances of higher concentration on one side of a semipermeable membrane show a net movement to the side of lower concentration until the concentrations are equal on both sides. When equilibrium is reached, movement across the membrane still occurs, but the number of particles going in one direction equals the number going the other way, thus yielding no net movement.
It should be noted that water (or another solvent) participates in this process. Net movement of water is usually in the opposite direction of solute movement, since a higher concentration of solute means, in effect, a lower concentration of solvent.
Fick’s law describes quantitatively the net movement of a substance across a semipermeable membrane where only diffusion is occurring:
Rate of movement = K(C1 – C2)
where
C1 = concentration on side with higher concentration
C2 = concentration on side with lower concentration
K = constant, which depends on nature and permeability of membrane, nature of solute and solvent, and temperature.
The less permeable the membrane to the solute or solvent, the lower the temperature, and the more viscous the fluid medium, then the longer will be the time required for equilibration. Therefore, diffusion tends to occur more rapidly through extracellular fluids than across cells. It should also be remembered that conditions for diffusion are markedly altered in a dynamic system such as the ciliary processes, in which blood is flowing rapidly, aqueous humor is constantly being formed and carried away, and other processes occur, such as those described below.
DIALYSIS AND ULTRAFILTRATION
In most biologic solutions, there exists a combination of salts, sugars, proteins, and other large molecules. Most biological membranes are permeable to water, salt, and some small organic molecules. However, these membranes are relatively impermeable to larger molecules, such as proteins.
If a solution of protein and salt is separated from either water or a less concentrated salt solution by a membrane permeable to the salt and water but not to the protein, then there will be a net movement of water to the protein side by diffusion, and a movement of salt away from the protein side. The protein, of course, cannot move across the membrane. This process is called dialysis (Fig. 5) and is utilized, for example, for removing unwanted salts and other toxic substances from the blood, using the peritoneum as the membrane (peritoneal dialysis), or by using a synthetic membrane such as that found in a dialysis machine (artificial kidney).
By the addition of a hydrostatic pressure on the protein side of the system, the exchange of salt and water can be accelerated. This process is called ultrafiltration and differs from dialysis only because the hydrostatic pressure changes the rate of movement of ions and slightly changes their final respective concentrations. The fluid formed by the process of dialysis is called a dialysate, and that formed by ultrafiltration is called an ultrafiltrate. Ultrafiltration is the process that occurs across capillary walls due to the higher pressure and higher protein concentration in the plasma as compared with the extracellular space (Fig. 6).
GIBBS-DONNAN EQUILIBRIUM
The salt does not distribute itself equally on both sides of the membrane. Since the protein carries an electrical charge (generally an overall negative charge at physiologic pH), the positive ions in solution tend to be bound to the negatively charged residues of the protein molecules. Thus, there is an excess of cations such as Na+ or K+ on the protein side of the membrane.
This unequal distribution is called the Gibbs-Donnan effect, and the quantitative relationships between the various ions (at least in simple systems) is predictable (Fig. 7). In order to maintain electrical balance, the total positive charges (e.g., number of Na+ and K+ ions) on one side must equal the total negative charges (e.g., sum of negative protein charges, Cl– ions, and any other negative ions). Furthermore, it has been shown that the final equilibrium concentrations of Na+ and Cl– on each side of the membrane are related according to the following equation1,30,31:
[Na+]1/[Na+]2 = [CL–]2/[CL–]1
Thus, if the aqueous humor were like extracellular fluid in most capillary beds of other parts of the body, we should expect to see a protein-free aqueous solution with ionic concentrations like those on side 2 of a Gibbs-Donnan ultrafiltrate of plasma. The Na+ and K+ concentrations should be less than plasma, and the Cl– and HCO3– concentrations should be slightly higher. Further, the actual values of the ratios of the concentrations of each respective ion in aqueous to plasma would conform to the values obtained experimentally when plasma is dialyzed against its own ultrafiltrate. In addition, no organic substance should be at higher concentration than in the plasma because diffusion and ultrafiltration can, at most, only equalize the concentrations of organic substances. These processes cannot promote an excess concentration on side 2 of the membrane.
Although aqueous humor resembles a dialysate of plasma in many ways, as will be seen, the ionic concentrations do not quite fit the Gibbs-Donnan predictions, and some nonionic substances have higher concentrations in aqueous than in plasma (Table 1). Such a condition can only occur in the presence of some active metabolic process.1,22
Table 1. Aqueous Versus Dialysate | |||||||||||||||||||||||||||||||||
---|---|---|---|---|---|---|---|---|---|---|---|---|---|---|---|---|---|---|---|---|---|---|---|---|---|---|---|---|---|---|---|---|---|
|
SECRETION
Secretion implies an active process that selectively transports some substances across the cell membrane. Because energy is consumed, substances can be moved across a concentration gradient in a direction opposite to that which would be expected by passive mechanisms alone. One example of this is the ability of the thyroid gland to accumulate iodide at up to 40 times the circulating plasma level.27,32 One would expect the iodide concentration in the plasma and the thyroid gland to be similar if only diffusion and ultrafiltration were operating. Aqueous humor exhibits increased ascorbate, lactate, and certain amino acid concentrations as compared with plasma, as a consequence of active secretion.1
Another way of testing for the presence of an active metabolic process is to apply specific metabolic inhibitors to the ciliary body and observe the effect on aqueous secretion. Ultrafiltration of fluid from the plasma to the posterior aqueous has been suggested to be responsible for approximately 70% of aqueous formation.27,33,34 However, the results of experiments where metabolic inhibitors have been used have shown conclusively that this is not the case. For example, systemic35 or intravitreal injection36 of ouabain (an inhibitor of the enzyme sodium potassium-activated adenosine triphosphatase [ATPase]—Na+/K+ ATPase) results in a decrease of up to 70% in aqueous formation, in a variety of species. The topical administration of vanadate (also a Na+/K+ ATPase inhibitor) lowers aqueous secretion in rabbits37,38 and monkeys.39 Ouabain as well as a number of other ion transport and channel blocking drugs significantly reduce aqueous humor formation in arterially perfused bovine eyes. Bumetanide (a specific inhibitor of Na-K-2Cl cotransport) and furosemide (a nonspecific anion transport inhibitor) reduce aqueous humor formation by 35% and 45% in bovine eyes in vitro. Similarly, 4,4′-diisothiocyanatostilbene-2,2′-disulfonic acid (DIDS, a probable inhibitor of the Cl-HCO3 exchanger, the Na-HCO3 cotransporter, and chloride channel) and 5-nitro-2-(3-phenylpropylamino)-benzoic acid (NPPB, a chloride channel blocker in nonpigmented cells) reduce aqueous humor formation by 55% and 25%, respectively, in bovine eyes in vitro.40 Also, catecholamines such as epinephrine, norepinephrine, isoproterenol and dopamine, that stimulate aqueous humor formation in humans also stimulate Na-K-Cl cotransport.41 If the greatest proportion of aqueous secretion was attributable to ultrafiltration, then this would not occur. In addition, Bill22 noted that the hydrostatic and oncotic forces that exist across the ciliary epithelium–posterior aqueous interface would favor resorption, not secretion, of aqueous humor. The ciliary process stroma has an oncotic pressure of approximately 14 mm Hg, because of its protein content. Because IOP in the healthy eye is maintained at approximately 15 mm Hg, a capillary hydrostatic pressure of greater than 29 mm Hg would be required to drive an ultrafiltrate. Capillary hydrostatic pressure in the ciliary stroma has been estimated to be 25 to 33 mm Hg.14 It has been calculated27 that a capillary pressure of greater than 50 mm Hg would be necessary to promote ultrafiltration as the major mechanism for the secretion of aqueous. The values of capillary hydrostatic pressure in the ciliary processes and a consideration of the hydrostatic and oncotic forces involved do not favor ultrafiltration as an important mechanism for aqueous humor secretion. Acetazolamide, and other specific inhibitors of the enzyme carbonic anhydrase, decrease the formation of aqueous by 40% to 60%.42,43,44 A reduction in temperature, which inhibits most active metabolic processes, also results in a decrease in aqueous formation, to a greater extent than would be expected if only diffusion were operating.1,45
Active transport systems usually exhibit a limit beyond which an increase in substrate concentration produces no further increase in transport. When this limit is reached, the system is said to be saturated. Thus, the fact that a transport mechanism for a substance can be saturated provides evidence of an active system. It has been demonstrated that by increasing the ascorbate concentration in plasma, a level of plasma ascorbate is reached above which no further increase in aqueous ascorbate concentration will occur. This provides evidence that the ascorbate transport system in the eye is saturable.
Several membrane active transport systems have been identified in the ciliary epithelium that are known to pump various substances against a concentration gradient, including Na+/K+ ATPase and amino acid membrane transporters (Fig. 8). There are also passive transport proteins specific for HCO3– and Cl–. Membrane-bound Na+/K+ ATPase is one important system involved in Na+ and K+ transport and is present mainly along the lateral cellular interdigitations of the nonpigmented ciliary epithelium.35,46,47,48,49,50,51 The transmembrane Na+/K+ transport protein is energized by the Gibbs free energy of hydrolysis of ATP, mediated by the ATPase enzyme intimately associated with it in the membrane. The ATP required for this process is derived predominantly from oxidative metabolism of glucose via the Krebs’ citric acid cycle. It is most likely that Na+ is pumped across the cell membrane and Cl– is passively carried with it in order to maintain electrical neutrality, although the relative rate of transport of Cl– is unclear.52 The Na+ pump induces a potential difference across the ciliary body, ranging from 6 to 10 mV. Measurements of the potential difference across the ciliary epithelia indicate that the aqueous is positive with respect to the stroma. The magnitude of this potential difference is reduced after poisoning with ouabain.53 These data are consistent with the hypothesis that Na+ is the primary mover and that active transport of Cl– is probably small in comparison to that of Na+.54 Interspecies differences in the aqueous-plasma ratios of Cl– (e.g., high in human, low in rabbits) might be explained by the relative proportions of Cl– actively transported.55 Transepithelial electrical measurements in the isolated rabbit iris-ciliary body indicate that Na+/K+ ATPase and HCO3– are required for active ion transport.56
However, recent studies suggest that active transport of Cl– ions plays an equally important role40,41,57 providing the driving force for aqueous humor formation.57 Shahidullah et al40 found that in the isolated bovine eye, aqueous humor is formed mostly by processes involving active secretion and chloride transport.
Recently, it has been discovered that water transport across many membranous barriers, including those in the eye, are facilitated by aquaporin (AQP) water channels.58 The AQPs are a family of water channels expressed in animals, plants, and lower organisms.58 There are at least 11 mammalian AQPs (AQP0 to AQP10) each of which is a small membrane protein approximately 30 kd in size. The eye expresses several AQPs at sites of fluid transport. AQP158,59 and AQP460 are expressed in the ciliary epithelium. AQP1-null mice have decreased IOP and aqueous humor production compared to normals.58
Histochemical studies have demonstrated a number of active enzyme systems in the ciliary epithelia. These include nucleotide phosphatases (especially ATPase, as mentioned previously), adenylate cyclase, and carbonic anhydrase, the enzyme that forms HCO3– (the body’s alkaline ion) from CO2 and H2O61,62,63,64,65 by the following reaction:
CO2+H2O→H2CO3→H+ + HCO3–
I II
Carbonic anhydrase catalyzes step I of the reaction and step II is an almost instantaneous ionic dissociation.66,67 The enzyme is localized in both the pigmented and the nonpigmented epithelial cells of the rabbit ciliary processes,68,69 and monkey and human pigmented and nonpigmented epithelial cells,70 most prominently in the basal and lateral membranes, but also in the cytoplasm. A variety of carbonic anhydrase inhibitors will reduce aqueous secretion, including acetazolamide,71 methazolamide, methoxazolamide, ethoxzolamide, dichlorphenamide,72,73 aminozolamide,74 trifluormethazolamide,75 6-hydroxyethoxzolamide,76 dorzolamide,77,78,79 brinzolamide,80,81,82 and several biscarbonylamidothiadiazole compounds.44 The site of action of these drugs is intraocular rather than systemic, because the unilateral injection of acetazolamide into the carotid artery lowers the IOP of only the ipsilateral feline eye.83 Precisely how carbonic anhydrase helps mediate aqueous secretion has been a long-standing debate. However, in recent years many advances in understanding of this system have been achieved. In the past, it was suggested that acetazolamide caused constriction of afferent iris or ciliary arteries, thereby decreasing ultrafiltration and hence aqueous humor formation.84,85 However, this view is no longer held. For example, Bill,86 using radiolabeled microspheres, concluded that acetazolamide had no significant effect on the blood flow in the intraocular tissues in the rabbit eye, and that it was unlikely that the drug had any effect on blood flow in the anterior uvea. It has now been irrefutably demonstrated that acetazolamide exerts its ocular effects via a reduction in aqueous secretion, mediated by a direct effect on the ciliary epithelial cells.26 The ciliary carbonic anhydrase system is now thought to function as described by Maren.87 Inhibition of the enzyme decreases the rate of Na+ and HCO3– transport into the posterior chamber by equimolar amounts, indicating a linkage of the accession of these two solutes into the posterior chamber of dogs and monkeys.88 Furthermore, inhibition of carbonic anhydrase lowers the aqueous Cl– concentration in primates and the HCO3– concentration in rabbits.24,89 The mechanism by which carbonic anhydrase activity is coupled to Na+ and HCO3– movement into the posterior chamber is still subject to much study. However, it is now known that the primary reaction, which occurs within the cytosol of the nonpigmented ciliary epithelial cells, is the proteolysis of water to yield OH– and H+ ions (Fig. 9). The hydroxide ions so formed react with CO2 catalytically (or noncatalytically when the enzyme is inhibited) to form HCO3–, which is passively transported to the aqueous humor, simultaneously with the active transport of Na+. The protons liberated from water pass into the blood circulation where they are buffered by proteins. The theoretical rates of flow of HCO3–, based on known constants and some assumptions regarding cell volume and pH of the secretory region, are shown at the bottom of Figure 9. The catalytic rate (i.e., when active carbonic anhydrase is present) is 7 μmol/min, and the uncatalyzed is 0.07 μmol/min.
The uncatalyzed rate is found experimentally by inhibiting the enzyme in vivo by a large dose of acetazolamide or similar compound. Such inhibition, when complete, should yield the uncatalyzed rate of HCO3– formation. Measurements obtained experimentally indicate that the observed inhibited rate (Fig. 9; 0.024 μmol/min) is not different from the calculated uncatalyzed rate of 0.07 μmol/min. Considering the assumptions about pH and cell volume, the difference between the calculated and experimentally measured inhibited rates of bicarbonate flow lies within acceptable limits of error. Inhibition of the flow of bicarbonate also leads to an inhibition of the flow of Na+. Several hypotheses have been put forward to explain this: (i) inhibition of carbonic anhydrase causes a decrease in HCO3– available for movement with Na+ to the aqueous side to maintain electroneutrality; (ii) a reduction in intracellular pH may inhibit Na+-K+ ATPase; and (iii) decreased availability of H+ produced by the reaction catalyzed by carbonic anhydrase decreases H+/Na+ exchange and reduces the availability of intracellular Na+ for transport into the intercellular channel.
A large excess of carbonic anhydrase has been found in the ciliary processes of all species studied. This has pharmacologic implications when one hopes to reduce aqueous secretion by inhibition of the enzyme. In order to achieve a clinically useful reduction in secretion and hence IOP, more than 99% of the enzyme must be inhibited. The sulfonamide carbonic anhydrase inhibitors have been in clinical use for glaucoma since 1955, when their action on the eye was discovered as an offshoot of their development as diuretics.73 They have also been extremely useful in research on aqueous humor formation, because they are highly specific, having no other actions at concentrations below 1 μM. One major compound is acetazolamide but many ophthalmologists have used methazolamide or related compounds, which have a greater diffusibility than acetazolamide, and that act on the eye in lower doses, thereby achieving the desired ocular effect but having lesser action on other systems in the body where carbonic anhydrase is present, such as the secretory epithelia of the kidney.42 Nonetheless, the systemic dose of sulfonamide carbonic anhydrase inhibitors necessary to significantly suppress aqueous secretion leads to undesirable side effects, even with methazolamide.90
In recent years topically applied carbonic anhydrase inhibitors have been developed for clinical use. Dorzolamide has been used since 199580,91,92 and brinzolamide since 1998.80 As a rule, the topically active carbonic anhydrase inhibitors have high lipid and water solubility, as well as high inhibitory potency and efficacy. Sufficient concentration is achieved in the ciliary processes via transcorneal, aqueous humor and iris absorption after instillation of a single drop.43,92,93 This can result in essentially as large a decrease in IOP as with the sulfonamides91,92,93 without the adverse side effects of oral acetazolamide. Dorzolamide has a high affinity for carbonic anhydrase and penetrates the eye well. However, some studies have found it to be a less effective at lowering IOP than oral acetazolamide.94 Brinzolamide’s effectiveness equals that of dorzolamide.80,81,95,96 However, both produce localized, albeit mild, side effects such as burning and stinging upon instillation and blurred vision.95,96 Brinzolamide produces less ocular discomfort than dorzolamide80,81,95,96 possibly due to the more physiologic pH of the preparation.80
In summary, aqueous humor is mainly a product of active cellular secretion requiring metabolic energy. Na+ and K+ are transported from plasma to the posterior chamber, with HCO3–, Cl– and water following passively to maintain electrical and osmotic balance. Other substances such as certain amino acids are also actively transported. Some other small molecules probably appear in the aqueous via diffusion or ultrafiltration.
The process begins with an ultrafiltrate of plasma in the extravascular spaces of the ciliary stroma. The ciliary epithelium (probably nonpigmented) then incorporates certain molecules and ions selectively for concentration and direct active secretion into the posterior chamber, while other substances are secreted passively.
BLOOD–AQUEOUS BARRIER
Large molecules such as proteins are present in the aqueous in only small quantities, even if their respective concentrations in the plasma are raised to high levels. In humans, normal plasma total protein levels are 6 g per 100 mL, compared to less than 20 mg per 100 mL in the aqueous, less than 0.5% that of plasma.97,98 Thus, a restraint in the free passage of many solutes from the blood vessels of the ciliary stroma into the aqueous humor exists. This constitutes one component of the blood–aqueous barrier,1,99 the anatomic correlate of which are the tight junctions between the interdigitating surfaces of the nonpigmented ciliary epithelial cells. Bill100,101 demonstrated that albumin and other proteins pass through the ciliary capillary walls at a much faster rate than they enter the aqueous humor and concluded that a barrier to these substances existed at a site other than the vessel walls. The intravenous injection of horseradish peroxidase into the monkey leads to a rapid filling of the ciliary stroma by the enzyme.102 The enzyme passes through the many fenestrations present in the blood capillaries of the ciliary body, since these have few tight junctions (similar to choroidal capillaries), and also fills the spaces between the pigmented cells. However, it is prevented from entering the aqueous by the tight junctions of the nonpigmented layer, between the cell apices. Comparable results have been reported in the mouse103,104 and in the rabbit.105,106 Numerous other studies have tested the blood–aqueous barrier using a number of different intravascular tracers designed to mimic the behavior of plasma-proteins.2 The conclusion of these studies is that tight junctions between the apicolateral surfaces of the nonpigmented epithelium of the ciliary body, and between the endothelial cells of the iris vasculature, prevent the passage of plasma proteins into the aqueous humor.102,103,104,105,107,108,109,110,111
The blood-aqueous barrier is not absolute. Water-soluble substances of medium molecular weight such as urea, creatinine, and certain sugars may penetrate at varying rates, but all are slower than their transit across capillary walls. Generally, the greater the lipid solubility coefficient of a substance, the greater its ability to penetrate the blood-aqueous barrier and pass to the posterior aqueous chamber.1,112,113,114 In addition, regional differences of permeability in the ciliary body have been noted. For instance, the epithelia of the anterior portions of the ciliary processes of the rabbit are less permeable than the epithelia of the pars plana.105
Substances such as mannitol are used clinically to reduce IOP. They function as hyperosmotic agents, by exploitation of the fact that they penetrate the blood–aqueous barrier only poorly but are distributed widely within the extracellular spaces of the body. Water is drawn from cells and the ocular fluids, balancing the high osmotic pressure induced in the extracellular space via the high concentrations of mannitol. The resulting loss of water from the eye leads to a reduction in the IOP.1 The effect of hyperosmotic agents is most pronounced in eyes exhibiting pathologically elevated IOP.115
Other examples of hyperosmotic agents include glycerin, urea, isosorbide, and ethanol. Urea was the first substance to be used for this purpose, however, it will slowly penetrate the blood–aqueous barrier, and hence the hyperosmotic effect of urea on the eye is shorter lived than that of mannitol. Ethanol is not used clinically because it penetrates the eye even more rapidly than urea and in the required doses has undesirable effects on the sensorium.
Certain antibiotics (e.g., chloramphenicol, cephalothin, and ampicillin) are known to penetrate the blood-aqueous barrier well, whereas others do so only poorly (e.g., penicillin, methicillin, erythromycin, and gentamicin).
A similar barrier to the passage of solutes exists in the retinal pigment epithelium, thus forming a blood–vitreous barrier between the vitreous and the blood capillaries of the choroid. Blood capillaries with tight junctions in their endothelia form further physiologic barriers (functionally similar to the blood–brain barrier) in the iris and retina.
SECONDARY AQUEOUS
The blood–aqueous barrier is fragile and may be disturbed by a variety of noxious stimuli. A corneal abrasion, paracentesis (withdrawal of a small volume of aqueous via a needle inserted into the anterior chamber),116,117,118,119,120 intraocular infection, uveal inflammation, intraocular surgery, and certain drugs, applied topically (such as nitrogen mustard or an anticholinesterase, e.g., echothiopate, diisopropyl flurophosphate [DFP], demecarium, neostigmine, or physostigmine), or delivered by intracarotid infusion, such as hyperosmotic agents (causing separation of the ciliary epithelial layers, opening of the blood–aqueous barrier, and severe, permanent damage to the pigmented epithelial cells121), are all capable of breaking down the blood-aqueous barrier and inducing changes in the aqueous humor composition (Table 2). Disruption of the blood–aqueous barrier has also been reported in the contralateral eyes of patients who have had cataract extraction and lens implantation surgery,122 and after argon laser trabeculoplasty.123 Severe damage to the blood-aqueous barrier occurs with cyclodestructive procedures used to treat advanced glaucoma and is evidenced by the prolonged or chronic presence of flare (the scattering of light upon slit-lamp examination by increased levels of protein in the anterior chamber).
Table 2. Factors Interrupting the Blood-Aqueous Barrier | |||||||||||||||||||||||||||
---|---|---|---|---|---|---|---|---|---|---|---|---|---|---|---|---|---|---|---|---|---|---|---|---|---|---|---|
|
After breakdown of the blood–aqueous barrier, the resultant aqueous produced is known as secondary or plasmoid aqueous.1,24,30,124 The most notable change is a marked increase in protein concentration. In this situation, the ionic composition of the aqueous approaches that of a simple dialysate of plasma, and substances that are normally barred from entering the aqueous now do so with ease. The unusually rapid rate of entry of substances such as fluorescein, Evan’s blue dye, albumin, or fibrinogen (which actually may allow the aqueous to coagulate) can be used as a diagnostic indicator of barrier breakdown.
Many of the processes that disrupt the blood–aqueous barrier also lead to vasodilation. Vasodilation can occur by means of an axon reflex (as may be seen after abrasion of the cornea), a sudden drop in IOP, or inflammation.125,126 In any case, vasodilation may be associated with loss of some of the tight junctions in the iridial vessels, which may help explain the breakdown of the barrier.125
In addition, it has been suggested that plasma proteins and other constituents can leak in a retrograde manner through Schlemm’s canal, and, therefore, account for the breakdown of the barrier after paracentesis.127 In this study, no changes in the permeability of the ciliary epithelium or iris to horseradish peroxidase were found after paracentesis, contradicting previous studies and conventional views of the mechanism of barrier breakdown.
Release of prostaglandins causes vasodilation and many other findings associated with inflammation. Evidence points to the possible involvement of this class of compounds with breakdown of the blood-aqueous barrier.124,128,129,130,131,132,133,134 Prostaglandins have been implicated in the irritative response after mechanical trauma to the eye, and cause miosis, vasodilation, release of protein into the aqueous, and elevated IOP. Prostaglandins applied topically to the eye in sufficiently high concentration cause breakdown of the tight junctions of the nonpigmented ciliary epithelium and increase the protein content of the aqueous humor, the highest levels of protein being found in the posterior chamber.135,136
Pretreatment with inhibitors of prostaglandin synthesis such as indomethacin or aspirin will inhibit breakdown of the blood-aqueous barrier, as well as some of the other manifestations of inflammation ordinarily induced by the aforementioned process.137,138,139,140,141 It may well be that prostaglandin release represents a final common pathway for the action of many different kinds of trauma and irritants. However, small doses of particular prostaglandins (especially prostaglandin F2α [PGF2α] and certain congeners) applied topically to the eyes of cynomolgus monkeys and humans result in a large increase in uveoscleral outflow, with a concomitantly large decrease in IOP, without clinically evident ocular inflammation.132,133,142,143,144,145,146,147 In the past decade, PGF2α analogues such as latanoprost,148,149,150,151,152,153 travoprost, and the prostamide, bimatoprost, have been approved for clinical use and are now some of the most commonly used drugs in the treatment of primary open-angle glaucoma (POAG) and ocular hypertension (see uveoscleral outflow section for greater detail).154,155
AQUEOUS HUMOR COMPOSITION
In the healthy eye, the aqueous humor has a refractive index taken to be constant at a value of 1.336.156 Because this index of refraction is lower than that of the cornea, there is a slight divergence of light rays as they pass the cornea–aqueous interface. Both the viscosity and density of aqueous humor are slightly higher than that of pure water, while the osmolality is slightly higher than that of plasma.30,157,158,159,160
The volume of the human anterior chamber is approximately 200 μL,3 while that of the posterior chamber is approximately 60 μL.1 This makes chemical analysis of the aqueous difficult because of the tiny volume of fluid as well as the relatively poor accessibility of the posterior chamber. Furthermore, it may account for the differences in values for the concentrations of many substances obtained by different investigators.
The greatest difference between aqueous and plasma resides in the very low protein concentration in the aqueous (Table 3), which is in the region of 0.5% that of plasma.97,98,157,158,159,160 However, the composition of protein in the aqueous is also different from that in plasma. The ratio of the levels of the lower molecular weight plasma proteins (such as albumin and the γ-globulins) to the higher molecular weight proteins (such as the α-lipoproteins and the heavy immunoglobulins) is much higher in aqueous in the normal healthy eye than in plasma.161 However, when the blood–aqueous barrier breaks down (as in uveitis), the composition and concentrations of protein in the aqueous are similar to that of plasma.98 The levels of immunoglobulin in the aqueous have been determined, both in the normal eye and in eyes in which the barrier has broken down.162,163,164 In the healthy eye, immunoglobulin (Ig) G is present at a concentration of approximately 3 mg per 100 mL,164 while IgM, IgD, and IgA are absent, presumably because of their larger molecular structure. In eyes with uveitis, the concentration of IgG increases, and IgM and IgA appear also. In the normal aqueous, trace concentrations of active complement C2, C6, and C7 globulins are also present.165
TABLE 3. Aqueous and Serum Protein Concentration | |||||||||||||||||||||||||||||
---|---|---|---|---|---|---|---|---|---|---|---|---|---|---|---|---|---|---|---|---|---|---|---|---|---|---|---|---|---|
|
There are also trace quantities of several components of the fibrinolytic and coagulation system present in the aqueous, with the exception of plasminogen and plasminogen proactivator, which are present at more significant concentration. Only trace quantities of the inhibitors of plasminogen activation are present in the aqueous,166 thereby ensuring that the aqueous outflow pathways remain free of fibrin. The aqueous humor of diseased eyes incorporates significant quantities of all of the major components of coagulation and fibrinolysis, giving rise to formation of intracameral clots; however, the composition of these clots is different from those that occur in blood vessels.24
The α and γ lens crystallins are also present in only small amounts in the aqueous humor of healthy eyes, although the concentration of these proteins increases in cataract.167,168
It has been calculated that in the normal healthy eye, the blood–aqueous barrier behaves as a semiporous membrane with a pore radius of approximately 10.4 nm. The protein concentration in the peripheral portion of the anterior chamber, close to the meshwork, may be much higher than in the more central region because of protein entry directly from the peripheral iris, as demonstrated in monkey and human eyes.169,170,171,172 Inclusion of serum in the perfusand of monkey173 or bovine174 eyes decreases resistance washout. The reduction in washout may be the result of interactions of particular serum proteins and not due to the general level of serum proteins.175
The concentration of amino acids, on the other hand, is frequently higher in the aqueous than in the plasma.176 In the canine eye, however, there is a lower concentration of amino acids in the aqueous than in the plasma.177 At least three transport systems for amino acids have been proposed in the eye,55 one each for the acidic, basic, and neutral groups. A statistical study of the covariation of the concentration of amino acids and related compounds in human aqueous suggested the existence of six transport systems in the ciliary epithelia: three independent mechanisms for neutral amino acids, and independent mechanisms for basic amino acids, acidic amino acids, and urea.178
The distribution of ions varies greatly amongst different species. For example, the monkey has a higher concentration of H+ and Cl– and a lower concentration of HCO3– compared to plasma. On the other hand, rabbit aqueous has a lower concentration of Cl– and H+ and a higher concentration of HCO3– compared to plasma.179 Active transport of Cl– across the feline isolated ciliary epithelium has been reported.52 The concentration of Na+ in the aqueous is almost the same as in the plasma in many species.1,30,180 However, the osmotic pressure of aqueous is slightly higher than that of plasma with respect to Na+, because of Gibbs-Donnan equilibrium.
Most species tested have very high concentrations of ascorbate and lactate in the aqueous. Ascorbate is actively secreted into the posterior chamber, and the secretion mechanism will only function in the presence of ATP and a Na+ gradient. The physiologic function of ascorbate remains to be elucidated; however, it is known to be concentrated by the lens epithelium181 and has been shown to have a protective effect against UV-induced DNA damage to lens epithelium.182 Ascorbate may function as an antioxidant, regulate the sol–gel balance of mucopolysaccharides in the trabecular meshwork, or partially absorb UV radiation183,184 because diurnal mammals have approximately 35 times the concentration of aqueous ascorbate than nocturnal mammals.185
The key oxidant in the aqueous humor, hydrogen peroxide, is normally present186 as a result of reactions of ascorbic acid and trace metals.187 Additional hydrogen peroxide and reactive oxygen species are generated by light-catalyzed reactions, metabolic pathways, and phagocytic or inflammatory processes.186,187 Hydrogen peroxide affects aqueous outflow in the calf perfusion system.188 Human trabecular meshwork cells exposed to 1 mmol of hydrogen peroxide show reduced adhesiveness to the extracellular matrix proteins fibronectin, laminin, and collagen types I and IV.189 Extensive and repeated oxidative stress in vivo may result in reduced TM cell adhesion, leading to cell loss that is identified as one of the major culprits in glaucomatous conditions.190,191,192,193
Lactate is produced as a result of the glycolytic degradation of glucose by both the ciliary body and the retina.194 It diffuses into the posterior chamber but is present at only marginally higher concentration than in the plasma at this site. However, it accumulates in the anterior chamber at considerably higher concentration than in the plasma.
Glucose, urea, and nonprotein nitrogen concentrations are slightly less than in plasma.1,30 Glucose is thought to diffuse into the aqueous, where its concentration is approximately 80% that of plasma. Glucose also diffuses into the cornea. Its concentration within the corneal endothelium is approximately half that in the aqueous. In diabetes mellitus, the aqueous concentration of glucose is increased. High glucose levels in the aqueous humor may increase fibronectin synthesis and accumulation in the trabecular meshwork and accelerate the depletion of trabecular meshwork cells. In bovine trabecular meshwork cells grown in high-glucose medium, fibronectin mRNA is significantly upregulated, fibronectin immunofluorescence is more intense, and relative amounts of fibronectin protein are significantly increased.195 Also the chemoattractant potential of fibronectin in aqueous humor is reported to play a role in trabecular meshwork cell loss in glaucoma.196
Oxygen is also present in the aqueous humor, at a tension determined to lie between 13 to 80 mm Hg, depending upon the method of measurement.197,198,199,200,201 The tension of oxygen in the aqueous can be decreased by topical epinephrine, possibly as a result of uveal vasoconstriction198 or by the wearing of polymethylmethacrylate (perspex) contact lenses, which by restricting the normal passage of oxygen across the corneal epithelium, cause corneal hypoxia and thus an increase in movement of oxygen from the aqueous, across the corneal endothelium.198
Berzelius202 first demonstrated the presence of lipids in bovine aqueous. Since then, sphingomyelin, phosphatidyl choline, and lysophosphatidyl choline have all been shown to be present in the aqueous,203 although at a concentration of less than 1 mg per 100 mL, because lipids are largely barred from entry to the aqueous by the blood–aqueous barrier.204
Other substances, such as corticosteroids,205 monoamine metabolites,206 Cr3+ ions,207 vitamin B12,208 sialic acid,209 and hyaluronic acid210 have all been found to exist in the aqueous of a number of different species. Hyaluronic acid covering the surfaces of the outflow pathways might prevent adherence of molecules to extracellular matrix components within the cribriform region and thereby prevent clogging of the outflow pathways.211 Knepper et al212 hypothesize that POAG is characterized by a decreased concentration of hyaluronic acid and increased turnover and downregulation of the hyaluronic acid receptor CD44 in the eye, which, in turn, may influence cell survival of TM and retinal ganglion cells. Corticosteroid regulation of IOP is proposed to occur via 11β-hydroxysteroid dehydrogenase (HSD)-1 expression that has been localized in the nonpigmented ciliary epithelium of human eyes.213,214,215 This enzyme catalyzes the conversion of cortisone to cortisol which, in turn, induces sodium and concomitant water transport into the posterior chamber, through epithelial sodium channels, including Na+-K+-ATPase,216,217 resulting in aqueous production. Levels of cortisol compared to cortisone in the aqueous humor are normally much greater than in the systemic circulation.218 However, long-term interactions of cortisol in the aqueous with glucocorticoid receptors in the trabecular meshwork, could contribute to increasing outflow resistance in individuals susceptible to steroid induced glaucoma (see section on trabecular outflow). Ingestion of the 11β-HSD antagonist carbenoxolone, decreased IOP in normal213 and ocular hypertensive214 human eyes. The role of the other compounds within the aqueous is not understood.
Transforming growth factor (TGF) β2 is a component of normal aqueous humor detected in many mammalian eyes219,220,221,222 and may play a role in glaucoma pathogenesis. The intrinsic activity of TGFβ2 is considered to be an important factor for the maintenance of the anterior chamber-associated immune deviation (ACAID).219,223 (see section on Aqueous Outflow: Other Agents for more details).
As the aqueous flows from the posterior chamber to the anterior chamber, changes occur. Nutrients diffuse into the lens, iris, and vitreous.224 Diffusion from the posterior aqueous into the vitreous is a major contributor to the existence of concentration gradients of low molecular weight substances in the vitreous.225 Waste products, such as lactate diffuse from the lens, iris, and corneal endothelium into the aqueous. Some exchanges of small molecules occur across the iridial vessels. Predictably, the chemical compositions of posterior chamber aqueous and anterior chamber aqueous are different.30 To complicate the matter further, some substances appear to be actively transported out of the eye. Paraaminohippurate (PAH), diodrast, and penicillin are examples of large anions that are actively transported out of the eye. The system appears to be similar to that occurring in the renal tubules. This active transport system can be saturated and can also be inhibited by low temperatures and probenecid.1,30 The nonpigmented ciliary epithelium has been implicated as the site of this active transport system within the anterior eye. Another independent transport system actively excretes injected iodide from the aqueous. This latter system resembles iodide transport mechanisms in the thyroid and salivary glands.226 The significance to the normal physiology of the eye of these outward-directed transport systems has not been established. With the discovery that prostaglandins may be actively transported out of the eye,227,228 some have suggested that such mechanisms may be useful to rid the eye of biologically active substances no longer needed, or which may even be detrimental.45 Their removal from the eye to the blood facilitates their excretion via the hepatic route. There are other outwardly directed ion-uptake mechanisms present in the eye. The anterior uvea of the rabbit eye, for example, accumulates the anions cholate, glycocholate, deoxycholate, chenodeoxy-cholate, iodipamide, and o-iodohippurate.229,230 At least one inwardly directed anion pump mechanism also operates—ascorbate is accumulated in the anterior chamber and lens, its concentration in the aqueous humor being approximately 20 times that in plasma.181 This may help protect the anterior ocular structures from oxidative damage. Bárány,231 making an analogy to the ion pump located at the renal peritubular cell border adjacent to the blood, which pumps simple cations from the blood to the kidney tubule, investigated whether there are any inwardly directed cation pump mechanisms from blood to aqueous. He concluded, however, that such mechanisms probably do not exist, at least within the rabbit eye. But outwardly directed cationic pump mechanisms have been reported. For example, iris–ciliary body preparations have been shown to accumulate the cation emepronium,232 although a later report233 questioned whether any other cations are actively eliminated from the eye.
AQUEOUS FLOW
The result of all of the biochemical processes previously discussed is the production of a quantity of fluid that circulates continuously. Many of the biochemical changes caused by diffusion occur as the fluid moves from the posterior chamber, through the pupil, around the anterior chamber, and into the outflow system. Superimposed on this bulk flow is the anterior chamber thermal circulation, which causes the aqueous closer to the cooler avascular posterior cornea (cooled additionally by evaporation of tears from the corneal epithelium) to move downward, whilst the aqueous closer to the warmer vascular anterior iris moves upward. In addition, movements of the eyes and head modify these flow parameters.
In the human eye, the rate of aqueous formation is approximately 2.5 μL/min, while that in the rabbit is approximately 3 to 4 μL/min. Because of the formation rate and aqueous chamber volumes in the human eye, approximately 3% of the posterior chamber volume and 1% of the anterior chamber volume are replaced per minute1,30 with the entire volume of the aqueous humor being replaced every 90 to 100 minutes.2 The more rapidly the aqueous is formed, the less is the potential for diffusional exchange with the ciliary processes, lens, anterior iris, and posterior cornea. However, within physiologic limits, changes in the rate of formation probably do not significantly affect the diffusional exchange. Furthermore, the rate of aqueous formation contributes to the regulation of IOP.
METHODS OF MEASURING RATES OF AQUEOUS FORMATION
In 1951, Goldmann234 first described a technique by which rate of flow of aqueous humor in the eye could be quantified. This technique was based on the measurement of the kinetics of unbound fluorescein in the plasma and concomitant fluorescence in the anterior chamber after intravenous injection.3 Thereafter, other investigators devised techniques for the determination of aqueous flow, generally involving a cannulation of the anterior chamber with a needle, thus permitting drainage of aqueous or infusion of a fluid at a known rate.235,236,237,238,239,240,241 For example, one can inject into the anterior chamber a known amount of dye, radioactively labeled substance, or large molecule, the concentration of which is easily measured. If the substance mixes rapidly with all of the aqueous, then repeated sampling of the fluid and measurement of the concentration provides an indirect measurement of bulk flow. The underlying assumption with this technique is that no change occurs in aqueous dynamics as a result of the injection or sampling technique. In practice such an assumption does not hold true, because the blood–aqueous barrier is breached by simple paracentesis.242 Furthermore, this technique assumes that none of the substance leaves the eye by diffusion or any other way except by direct bulk flow (which includes outflow via the uveoscleral pathway).
Later still, other techniques more applicable to the measurement of aqueous formation in the human eye were devised, which did not involve any invasive procedure.243,244,245,246,247,248,249 These methods, discussed in the following section, fall essentially into one of two categories: (i) measurement of the rate of appearance or disappearance of a chemical substance from the aqueous or (ii) derivation of the aqueous formation rate from a mathematical formula (to be discussed) after obtaining measurements of the IOP, episcleral venous pressure, and resistance to aqueous outflow.1,30 Each method incorporates intrinsic advantages and disadvantages, and specific sources of error. However, in spite of this, there exists good agreement for the values of aqueous formation obtained, and in several different species.
MEASUREMENT OF FORMATION BY THE RATE OF APPEARANCE OR DISAPPEARANCE OF A CHEMICAL SUBSTANCE FROM THE AQUEOUS
It is possible to inject a substance such as PAH or fluorescein into the bloodstream and maintain a high concentration there. Over a period of several hours, a small but measurable amount diffuses into the aqueous humor and reaches an equilibrated concentration. The injection is then stopped, and the level of the fluorescein or PAH in the blood subsequently declines rapidly, owing to renal clearance. The concentration of the substance in the aqueous, however, remains relatively constant over a short period of time, after which it starts to decrease, as fresh aqueous is formed not incorporating any PAH or fluorescein, because of low plasma levels. The rate of fresh aqueous formation can be calculated by measuring the declining concentration either by sampling the aqueous first in one eye, then later in the second eye, as in the case of PAH, or by optical means using fluorophotometry, as in the case of fluorescein (Fig. 10). Bárány and Kinsey250 developed this technique for PAH, and it was extended for use with fluorescein.248,251,252,253 The technique using fluorescein has been modified by dropping the fluorescein topically onto the eye rather than by injecting it.254,255 The dye in this case gains access to the anterior chamber via corneal absorption. There are, however, inherent problems with this approach, specifically, the problem of measurement of fluorescence in the anterior chamber and cornea, and the deduction of aqueous flow from the change in fluorescence over time.3 The first problem was solved by the construction of a slit-lamp fluorometer.256,257 The second was addressed by a number of investigators who devised several new experimental approaches.55,250,258 Thus, despite the drawbacks of fluorophotometry using topically applied fluorescein, the technique has become the gold standard in studies involving the human eye. Jones and Maurice248 realized that the corneal stroma could serve as a depot from which fluorescein could be introduced slowly into the anterior chamber. This method clarified the important role of the cornea in affecting the kinetics of topically applied drugs and tracers. Maurice’s technique is now used most frequently for the measurement of the rate of aqueous formation in the human eye. The method involves the application of fluorescein topically to the eye, which subsequently penetrates the corneal epithelium and enters the stroma. Fluorescein is not metabolized by the eye and thus can disappear in only three ways: (i) rediffusion through the corneal epithelium and loss with the tears, (ii) lateral diffusion into the limbal tissue, or (iii) penetration of the endothelium and entrance into the aqueous humor, from where it is washed away by flowing aqueous, or is lost by diffusion into the iris (approximately 10% of fluorescein in the aqueous is lost by this route in the human eye).259 The third pathway of movement of fluorescein from the corneal stroma offers the least resistance, and thus is the major pathway of loss of fluorescein from the stroma.
The advantages of Maurice’s technique are that it is safe, repeatable, and objective. Studies of the human eye can span 18 to 24 hours after application of a single dose of fluorescein. The procedure disturbs the eye minimally, and the subject need not be constrained during the interval of measurement. Furthermore, the technique is reliable even when the rate of flow is not constant.3
In one variation of this technique, fluorescein is introduced into the stroma simply by applying drops topically to the inferior fornix of the conjunctiva.254,260 A waiting period of 6 hours or more allows the dye to become more uniformly distributed within the stroma. In another variation, fluorescein is applied by iontophoresis.248 This procedure involves the introduction of fluorescein into the anterior chamber by forcing it through the cornea via the application of a small electric current. Fluorescence is measured in the stroma and in the anterior chamber at the beginning and end of an interval. Flow is the clearance of the dye during the interval minus the diffusional loss. The technique, however, is not applicable to eyes that lack an iridolenticular barrier between the anterior and posterior chambers. Furthermore, the technique measures only that portion of secreted aqueous that passes into the anterior chamber.3
McLaren261 developed a technique of measurement of aqueous flow based on flare. Flare (pathologic scattering of light resulting from the presence of protein in the aqueous resulting from inflammation and breakdown of the blood–aqueous barrier) was induced by argon laser photocoagulation of the iris in rabbits, and a scanning ocular spectrofluorophotometer was used to measure scattering in the anterior chamber. This method was used to study changes in aqueous flow over the diurnal cycle. A technique for the measurement of aqueous flow using corneal and vitreous depots of fluorescein in the rabbit eye has also been described.262,263 It may be concluded that (i) movement of water into or out of the vitreous can cause large changes in the rate of movement of dye from the vitreous to the anterior chamber and can make interpretation of the vitreous method ambiguous and (ii) the vitreous method is probably superior for measuring sustained changes of the rate of aqueous flow over at least 10 hours, or perhaps several days, but it cannot be reliably used for measuring changes over shorter periods.
Similar types of studies have been performed using iodide.264 In all cases, it is assumed that the amount of substance leaving the eye by alternative routes is negligible compared with the dilution by fresh aqueous.
By using the method of Maurice and introducing fluorescein by iontophoresis, a value of 2.48 ± 0.17 μL/min (mean ± standard error of the mean [SEM]) was calculated for aqueous flow in the human eye.248 Holm245 carried this method one step further. After forcing fluorescein into the anterior chamber, the fluorescein is carefully mixed by rapid eye movements. Then, as fresh aqueous humor not containing fluoresecein is produced, it forms a small, clear, growing bubble at the pupillary border. Multiple slit images are projected onto the bubble and photographed. The volume of the bubble can be estimated by measuring the deviation of each slit as it passes over the bubble and integrating the area of all the deviations. Two sets of photographs are taken 15 seconds apart in order to calculate the rate of change. However, the pupil must be miotic (constricted) for this technique to work, and a miosis is induced by topical application of pilocarpine, which may stimulate aqueous flow slightly.265 Furthermore, the production and maintenance of the bubble of clear aqueous is technically difficult. For these reasons, Holm’s technique of iontophoresis has not gained widespread popularity in studies of aqueous humor secretion, either in the human or animal eye, although the results that have been obtained using this method agree closely with those obtained using Maurice’s technique of fluorophotometry.3
In all the techniques whereby a fluorescent dye is used to measure aqueous flow, there are other potential confounding factors, such as binding of a proportion of the dye to ocular and/or plasma proteins, that must be considered.266 However, under normal circumstances these factors do not compromise the measurements.
MATHEMATICAL DERIVATION OF AQUEOUS FORMATION RATE
The other major types of investigational tools used to estimate aqueous formation involve physical measurements. These methods depend on the following algebraic manipulation of the modified Goldmann equation describing IOP in terms of episcleral venous pressure (Pe), aqueous flow (Fin), trabecular outflow facility (Ctrab), and uveoscleral outflow (Fu):
IOP = Pe + ((Fin-Fu)/Ctrab))
thus
IOP-Pe = ((Fin-Fu)/Ctrab))
and
Fin – Fu = Ctrab (IOP – Pe)
therefore
Fin = Ctrab (IOP – Pe) + Fu
In order to determine Ctrab and Fu, the eye in a living anesthetized animal may be cannulated with a needle and perfused with a solution of mock aqueous humor267 incorporating radiolabeled albumin (see later in this chapter).268,269 A value for IOP can be obtained by tonometry, or (in experimental animals) cannulation of the anterior chamber with connection via tubing to a pressure transducer. An estimate of Pe can be obtained in experimental animals by direct cannulation of an episcleral vein, or by measurement of the pressure necessary to completely collapse an episcleral vein.270 (In practice these procedures would be repeated several times and a mean value calculated, because Pe is not constant throughout the entire episcleral venous system). By substitution into the above equation, Fin may be determined. Fin can also be calculated by determination of the difference in radioactivity of a perfused radiolabeled solution before entering and after leaving the anterior chamber, the difference being related proportionally to total aqueous flow. These approaches, however, are applicable only to experimental animals; their invasive nature makes them unsuitable for use in the human eye.
Another method for estimating the rate of aqueous flow involves the use of a perilimbal suction cup.85,271 The perilimbal suction, theoretically, occludes the outflow channels of the eye, and thus IOP rises. The rate of increase in IOP depends not only on the rate of formation of aqueous humor but also on the elasticity or distensibility of the eye. Unfortunately, there is a question as to whether this method may also alter the blood flow to the ciliary body, thus possibly having an effect on aqueous formation. Furthermore, as the pressure rises in the eye, the rate of inflow of aqueous humor declines, a phenomenon known as pseudofacility. In addition, the suction cup may not totally close off the drainage of aqueous. Nevertheless, Galin,271 using this method, calculated rates of aqueous formation that compare well with other methods.
It is apparent that each of the foregoing methods has inherent errors and assumptions, yet despite this, the results indicate reasonable agreement among the various methods. The study of these methods has added much to the understanding of the factors that influence aqueous formation. In fact, it is not so much the absolute values themselves that are important but, rather the ability to compare changes in values for aqueous formation under different conditions that has been most informative.
Of all of these methods, however, noninvasive fluorophotometry has become the method of choice for the determination of the rate of flow of aqueous.
FACTORS AFFECTING AQUEOUS FORMATION
The rate of formation of aqueous is not constant. Variations in the formation rate occur hourly and daily.3 Knowledge concerning the cause(s) of this variation is particularly sparse. It is likely that many diverse physiologic systems, including the central nervous, endocrine, and cardiovascular systems, as well as changes in metabolic activity, all influence the production of aqueous.
Aqueous secretion also exhibits a diurnal cycle.1,24,272 It is hypothesized that the diurnal cycle of aqueous humor formation is regulated in part by a factors such as circulating catecholamines, epinephrine and norepinephrine, that have a circadian rhythm and partly by a factor that depends on the activity of the subject.272 Further, the magnitude of the fluctuation in flow rate differs among different individuals.
The ciliary body is innervated by nerves arising from the long posterior and short ciliary nerves, which run parallel to the long posterior and short ciliary arteries. These nerve fibers are of both the myelinated and nonmyelinated variety. Parasympathetic fibers originate in the Edinger-Westphal nucleus of the third cranial nerve, run with the inferior division of this nerve in the orbit, and synapse in the ciliary ganglion.225 Sympathetic fibers synapse in the superior cervical ganglion and are distributed to the muscles and blood vessels of the ciliary body. Numerous unmyelinated nerve fibers surround the stromal vessels of the ciliary processes; these are most likely noradrenergic and subserve vasomotion.24 Sensory fibers arise from the ophthalmic division of the trigeminal nerve and enter the ciliary body, but their distribution and function have not been well studied. However, despite the distribution of nerves to the ciliary body, little evidence for innervation of the ciliary epithelium itself has yet been found.4 Wetzel and Eldred273 found that some of the dendritic processes from peptidergic amacrine cells in the retina of the turtle formed a dense circumferentially oriented nerve fiber plexus and that collaterals from this plexus projected into and innervated the nonpigmented ciliary epithelium in the pars plana region of the ciliary body. The authors suggested that these peptidergic amacrine cells may be involved in the control of aqueous inflow.273 It has also been found that stimulation of the ciliary ganglion or application of cholinergic agents, in nonprimates, will lead to an increase in aqueous formation, even in the isolated, perfused feline eye.85 Additionally, in this model, sympathetic activity was reported to suppress aqueous secretion. Also, in the bovine perfused eye model,274 delivery of the adrenergic agonist terbutaline via the intra-arterial route to the ciliary body was found to decrease the rate of aqueous secretion.275
Results obtained with isolated perfused eyes of nonprimate animals, however, may bear little relationship to the in vivo physiologic situation reported in humans and subhuman primates. For example, adrenergic agonists are reported almost exclusively to increase aqueous secretion in primates. Topical epinephrine, norepinephrine, or isoproterenol stimulate secretion in the monkey eye,276,277 and topical epinephrine or terbutaline stimulate aqueous secretion in the human eye.278,279 Whether these processes work at the vascular level to change vascular tone or permeability or their effect is dependent on ciliary epithelial cell surface neurohumoral receptors associated with adenylate cyclase and/or guanylate cyclase activity has not yet been completely elucidated; however an amassing body of evidence points to the latter.275,280,281,282,283,284,285,286,287,288,289,290,291,292,293,294,295,296
The α2-adrenoceptor agonists apraclonidine and brimonidine are reported to lower aqueous secretion and IOP in cats, rabbits, monkeys,297,298,299 and humans.300 The effect is thought to be mediated via a postjunctional or nonjunctional α2 receptor present on the membrane of the nonpigmented ciliary epithelium299 but may in part be centrally mediated via imidazoline receptors in some species.301 The effect in monkeys is not abolished by superior cervical ganglionectomy,299 indicating that intact sympathetic innervation is not required for the drugs to lower secretion and IOP. However, intact sympathetic innervation is required for a response to the α2-adrenoceptor agonists clonidine in rabbits302 or brimonidine in cats and rabbits.297 The lack of aqueous flow suppression by brimonidine in pentobarbital-anesthetized monkeys may reflect the suppression of neural and humoral catecholaminergic tone by this anesthetic,303 especially relative to ketamine, which elevates such tone.304 Timolol, a nonselective β-adrenergic antagonist, suppresses aqueous flow in both eyes of ketamine305 but not pentobarbital-anesthetized306 monkeys that have undergone unilateral superior cervical ganglionectomy, suggesting that the relevant β-receptors may be nonjunctional rather than postjunctional and that sympathetic neural tone to the ciliary body may play relatively little role in regulating aqueous humor formation in the monkey. It is thus not entirely clear to what extent aqueous secretion is under neuronal versus humoral adrenergic control.
The effects of cholinergic drugs on aqueous humor formation and composition, and on the blood–aqueous barrier, are unclear, with conflicting results arising from various studies. In general, cholinergic drugs cause vasodilation307,308,309 resulting in increased blood flow to the iris, ciliary processes and ciliary muscle.309,310 The presence of flare (Tyndall effect, indicating increased protein concentration) and/or the detection of cells in the aqueous humor by biomicroscopy indicates that these agents can also cause breakdown in the blood-aqueous barrier.311 Pilocarpine increases barrier permeability to iodide264 and inulin.312 Cholinergic drugs may alter the aqueous humor concentration of inorganic ions313 and the movement of certain amino acids from the blood into the aqueous humor, and may also influence the outward-directed transport systems of the ciliary processes.314,315 Under certain conditions, pilocarpine may increase pseudofacility.316,317 Cholinergic agents or parasympathetic nerve stimulation have been reported to increase, decrease, or not alter aqueous humor formation rate and to slightly increase the episcleral venous pressure.265,317,318,319,320,321,322,323,324 The minimal effect on the rate of aqueous humor formation and episcleral venous pressure are clearly not responsible for the drug-induced decrease in IOP that results from cholinergic therapy.
Aqueous formation varies directly with the blood pressure in the internal carotid-ophthalmic arterial system in the primate277 but only when blood pressure is altered artificially to a physiologically abnormal extent. For example, ligation of the internal carotid artery causes a profound drop in aqueous secretion. Formation is not significantly altered however by changes in blood pressure within the normal physiologic range for any given species. Aqueous formation rate diminishes slightly with age. After the age of approximately 10 years, formation declines by 3.2% to 3.5% per decade.3 The mechanism underlying this decrease is unknown. An age-dependent loss of ciliary epithelial cells has not been described. Some authors have demonstrated a 5.8% loss of trabecular cells per decade in humans,190,325,326 while others have reported a loss of corneal endothelial cells at the rate of 3.5% per decade.327 Brubaker3 suggested that the age dependency of the population of ciliary epithelial cells should be studied, because if it is found that aqueous formation parallels the number of secreting cells, it would suggest that although aqueous formation may depend on neuronal or hormonal stimulation, the normal rate of formation may depend on cell count. Alternatively, the decline in aqueous formation could be a result of the changes observed in the fine structure of aging ciliary epithelial cells.328 Hypothermia leads to a decrease in aqueous formation, for example, a drop in body temperature of 7°C (about 19%) leads to approximately a 50% reduction in secretion, reflecting the deactivation of metabolic processes necessary to maintain active secretion.45
The ultrafiltration component of aqueous humor formation is pressure-sensitive, decreasing with increasing IOP. This phenomenon is quantifiable and is termed pseudofacility, because a pressure-sensitive decrease in inflow appears as an increase in outflow facility when techniques such as tonography and constant pressure perfusion are used to measure outflow facility.237,238,316,329,330,331,332,333 Although some sensory nerve endings exist in the ciliary body, they do not appear to be of the pressure-sensitive variety.4 The initiating event of this pressure-induced response of the ciliary processes remains obscure. Bill and Bárány237 reported that an artificially induced rise in IOP caused a reduction in secretion. This has been confirmed in the monkey.334 An intracameral injection of erythrocytes yielded a partial blockade of the trabecular meshwork, and hence an elevation in IOP. This led to a suppression of aqueous secretion, corresponding to 0.06 μL/mm Hg per minute increase in pressure. Therefore with a normal secretion rate in the pentobarbital anesthetized cynomolgus monkey of approximately 1 μL/min,335 an increase in IOP of 20 mm Hg should theoretically suppress aqueous secretion altogether. However, this does not in fact occur. It is well established that secretion continues, even against very high pressures. Increased IOP does decrease the blood flow in the ciliary body and may decrease secretion in this manner.315,330,336 In addition to the acute effect of elevated IOP, some patients in the late stages of glaucoma may show hyposecretion and even have normal pressures despite almost totally occluded outflow channels.337 It is not known if this is due to the same mechanism as the acute type of pressure-related hyposecretion. There is evidence, however, that much of the perceived reduction in aqueous secretion in response to increased IOP is caused by measurement artifact237,238,268,315,334,338 and that the real magnitude of pseudofacility in the monkey is less than 0.02 μL/mm Hg per minute, or less than 5% of total facility.238,339
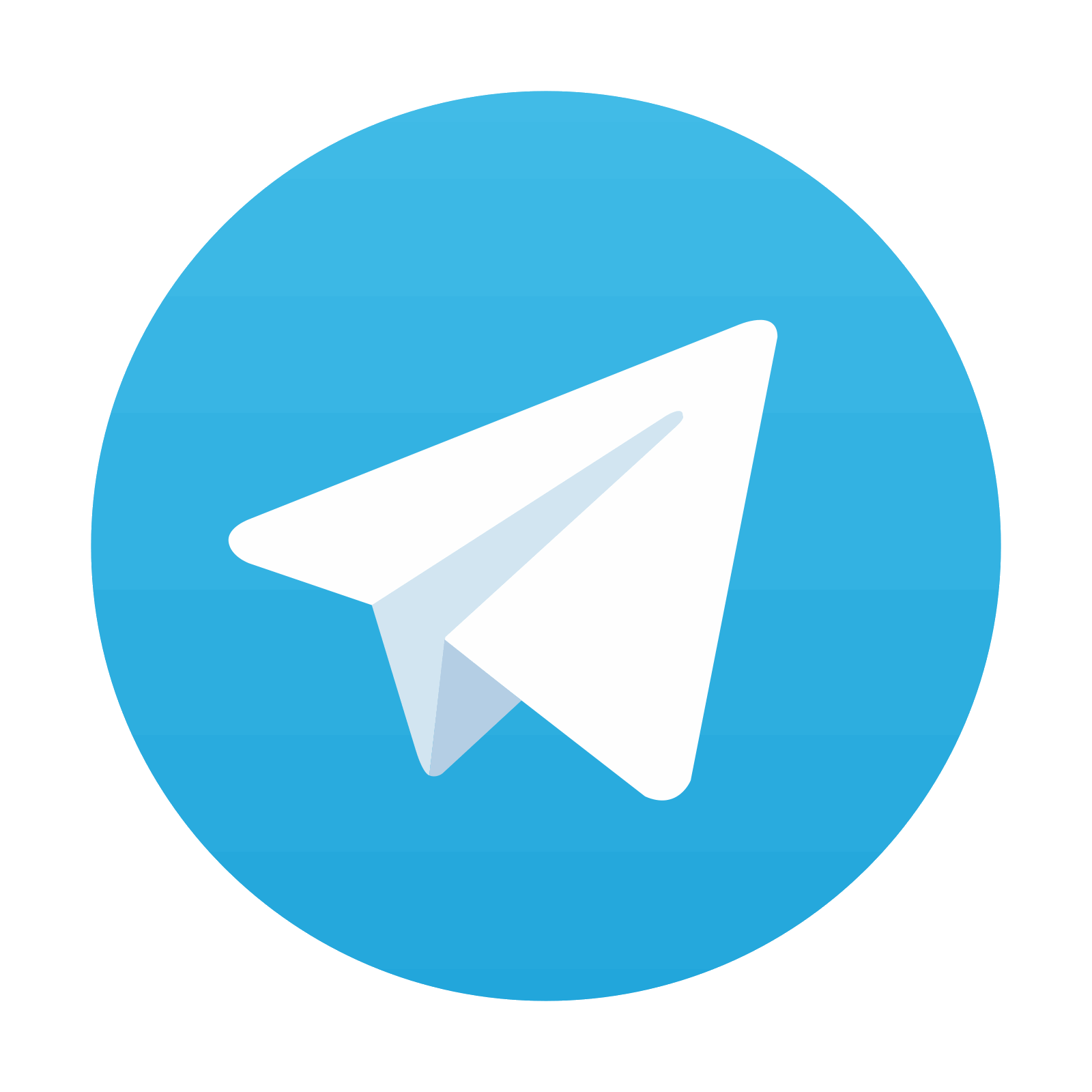
Stay updated, free articles. Join our Telegram channel
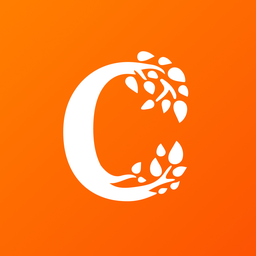
Full access? Get Clinical Tree
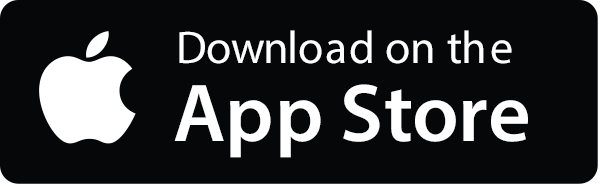
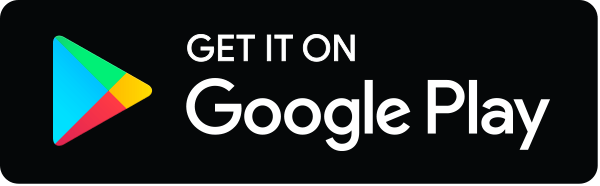