Applications of Optical Coherence Tomography in the Diagnosis and Management of Glaucoma
Irina V. Koreen
Chirag Gupta
Larry Koreen
Sanjay Asrani
Neelakshi Bhagat
Joshua D. Stein
BACKGROUND
HISTORY OF OCT
Optical coherence tomography (OCT), introduced in 1991 by Huang et al,1 is a technique that allows noncontact cross-sectional imaging of biologic tissues in vivo with micrometer-scale resolution. Since its inception, OCT has been used in various fields in medicine2,3,4,5 and in biologic research.6 However, the field where it has had the most clinical utility to date and where it made the fastest transition to routine clinical practice is ophthalmology. In vivo imaging of the posterior segment of the eye was first reported in 1993,7,8 and anterior segment imaging using OCT in 1994.9 Clinical trials demonstrating the utility of OCT in imaging ocular pathology were reported several years later,10,11 and the first commercially available OCT system was introduced by Carl Zeiss Meditec Inc. (Dublin, CA) in 1995. Since then, several generations of the instrument have been developed: OCT2, also known as OCT 2000; OCT3, also known as Stratus OCT; Spectral Domain OCT (SD-OCT); and Visante OCT and Slit-lamp OCT (SL-OCT) for anterior segment imaging. In 2008, the most widely used models were the Stratus OCT for posterior segment imaging and the Visante OCT for anterior segment imaging. SD-OCT has greatly improved resolution and image acquisition speed, which permits detailed three-dimensional imaging of the posterior pole, and is rapidly gaining popularity since its commercial introduction in 2007.
COMPARISON OF OCT WITH OTHER OCULAR IMAGING MODALITIES
The resolution of Stratus OCT (∼10 μm) far exceeds that of computerized axial tomography (CT, 300 μm),12 magnetic resonance imaging (MRI, 300 μm),13 confocal scanning laser ophthalmoscopy (CSLO, 60 μm),14 standard B-mode ultrasound (200 μm),15 and high-frequency (100 MHz) ultrasound (20 μm).16 SD-OCT has an even higher resolution of approximately 3 μm, improving the visualization of the layers of the retina, retinal pigment epithelium (RPE), and the choroid.17,18
The near-infrared wavelength light used in OCT imaging appears as a barely visible thin red line to the patient, enabling use of the instrument to be a nonpainful, noncontact and noninvasive procedure. Furthermore, the retinal exposure to the infrared light at 800 nm wavelength, with a power level of approximately 200 uW, is well within the exposure limit recommended by the American National Standards Institute, an indication that the technology is safe.19 However, unlike instruments such as ultrasound, OCT requires a relatively clear media to assess the posterior segment. For example, the utility of OCT in the presence of corneal edema, dense cataract, or vitreous hemorrhage is limited.
The technology allows clinicians to quickly capture clinical information. A standard 6-mm Stratus OCT scan takes 0.5 to 1.5 seconds to complete. The fiberoptic technology used in OCT allows for a very compact instrument that can easily be incorporated into a standard ophthalmology office, similar to an ultrasound scanner.
PRINCIPLES OF OCT
PHYSICAL PRINCIPLES
The principal components of a time domain OCT (TD-OCT) scanner include a fundus camera, a light source, a beamsplitter, a slitlamp biomicroscope (sample light path), a reference light path with a reflecting mirror, and a detection module (photodetector, demodulator, digitizer, computer). Low-coherence light at approximately 800 nm with wavelength bandwidth of 20 nm is emitted from a superluminescent diode, which is a semiconductor light source, and divided by the beamsplitter into the sample and reference light paths. The light in the sample path is focused on the retina through a slit lamp biomicroscope equipped with a 78D focusing lens positioned about 1 cm from the eye. The light in the reference path is reflected from the reference mirror as the mirror continuously moves in the axial direction, changing the length of the reference path. The light reflected from the eye in the sample path (the backreflected and backscattered “echoes”) is combined with the light simultaneously reflected from the reference path to produce an interference pattern that is measured by the photodetector, digitized, and stored on the computer.1
The sample and reference light paths produce an interference pattern only if their path lengths are within one coherence length of each other. The presence of interference at various reference mirror positions is used to calculate the axial distance within the ocular tissue of each light echo, also known as the echo time delay. The interference pattern is also used to calculate the amplitude of the light echoes. Combining the echo amplitude and time delay, the computer calculates and plots the logarithm of the light backreflectance and backscattering of the ocular tissue as a function of axial distance (depth) in the tissue. This line plot is displayed as either a grey-scale or false-color image. The scan is then repeated by translating the light beam laterally across the retina to produce a stacked image, a retinal tomogram (Fig. 42-1). Each tomogram typically consists of 512 axial scans (A-scans) spanning 6 mm. The displayed image is elongated in the axial dimension (vertically) for ease of viewing.
The axial resolution is determined by the coherence length (or wavelength bandwidth) of the light source. The transverse resolution is determined by the focused spot size of the OCT beam on the tissue being examined, which is in turn affected by the diffraction properties of the tissue and the focusing parameters used.1 The focused beam size on the retina in TD-OCT systems is approximately 20 μm, leading to a transverse resolution of 20 to 25 μm.1,20 The newer SD-OCT systems do not rely on a reference light path with a continuously translating mirror. This allows much more rapid image acquisition and improved resolution.
The technical details of anterior segment imaging with OCT differ significantly from those of retinal imaging. Although the anterior segment can be imaged using a retinal OCT scanner, because of scattering of the 830-nm wavelength light by scleral tissue at the limbus, the image quality of structures such as the iridocorneal angle is inadequate.9 However, the use of a 1310-nm wavelength light source has enabled imaging of the anterior segment with certain prototypic OCT machines.21 Using this wavelength with SD-OCT, a more detailed visualization of the anterior segment structures is achieved.22,23 The 1310-nm wavelength penetrates the sclera and enables imaging of the iridocorneal angle structures, as well as high-speed scanning (as high as 4,000 axial scans per second) made possible by the higher permissible exposure limit of 1,310 nm wavelength light.21 While this greatly enhances visualization of anterior segment structures, dispersion of light by the iris pigmented epithelium limits imaging of structures located behind the iris.
IMAGE ACQUISITION AND PROCESSING
During scan acquisition using Stratus OCT, beam positioning on the retina is monitored by the operator using an infrared-sensitive charge coupled device (CCD) video camera, and the beam is focused by axially translating the 78D focusing lens. There are options for both internal (test eye) and external (contralateral eye) target fixation for the patient. The acquisition time for a single high-resolution tomogram is approximately 1.5 seconds. Despite such quick acquisition speed, eye motion resulting from pulse pressure, microsaccades, tremors, and changes in fixation may cause image artifacts. Lateral eye movements are not compensated for in the processed image, so good fixation is essential.
The Stratus OCT software has algorithms for boundary detection of the retinal layers and various scan acquisition protocols. Line scans include a single line of variable length and direction, a series of equally spaced parallel lines, a set of two perpendicular lines, or a set of radial lines equally spaced through a common center. The radial line scans are particularly useful for macular thickness and optic nerve head (ONH) mapping. There are also several options for circle scans: a single circle of arbitrary size, a proportional circle that allows one to match the circle size to the size of the optic disc, and a series of concentric circles with customizable ratios. The circle protocols are most useful for retinal nerve fiber layer (RNFL) thickness analyses.24
Posterior segment OCT tomograms are generally displayed in false-color format. The most backreflecting and backscattering layers are displayed in a spectrum of red to white, layers with intermediate backscattering properties appear green to yellow, and the least backscattering and backreflecting layers are displayed in a spectrum of blue to black.
The image acquisition is similar for anterior segment OCT. Linear scans are most often used to obtain a cross section of the cornea and anterior chamber. The images are displayed in a grey-scale format. Volumetric scans of the anterior chamber are also possible with certain prototypic systems.23
INTERPRETATION OF IMAGES
NORMAL FINDINGS AND STRUCTURES
Prior to detailed discussion of the features of OCT tomograms, it is essential to understand that different tissue layers can be distinguished only on the basis of a contrast in backscattering and backreflectance properties between adjacent layers. Pathologic changes that do not alter the tissue organization or the backscattering and backreflectance properties of the tissue are not detectable by OCT. Furthermore, as in ultrasound, tissue components with a significant amount of backscattering, such as blood vessels, hemorrhages, and hard exudates, cause shadowing of the deeper structures by diminishing light transmission, and make them difficult to image.
Optic Nerve/Retinal Nerve Fiber Layer
Using OCT, the thickness of the RNFL can be measured.25 OCT sections through a healthy optic disc show increased peripapillary RNFL thickness in the superotemporal and inferotemporal regions, which corresponds to normal bundling of the ganglion cell axons in these regions. The nerve fiber layer measurement is performed by segmentation of the innermost reflective layer of the retina using software algorithms. The two typical software strategies are as follows: Fast RNFL involves three superimposed circular measurements along a peripapillary circle, and the average of the three measurements is reported as thickness in microns; six-ring peripapillary concentric measures of nerve fiber layer is performed by measuring the RNFL along six increasing-diameter concentric peripapillary rings, and the result is displayed as a distribution map of the nerve fiber layer.26
Retina
When imaging the retina by using OCT, large-scale anatomic features—such as the fovea, optic disc, and retinal curvature—are easily recognizable by their morphology (Fig. 42-1). Retinal blood vessels cause shadowing of the deeper retinal layers on the tomogram. One can easily see the inner boundary of the retina as the interface between the weakly backscattering vitreous, which appears black, and the highly backscattering RNFL, which appears red. The outer boundary of the retina is also easily detectable as the highly backscattering band composed of the RPE and choriocapillaris (Fig. 42-1). With SD-OCT, more detailed identification of the layers of the retina, RPE, and choroid is possible (Fig. 42-2). For example, this device allows resolution of the delicate photoreceptor inner and outer segments, the RPE, choriocapillaris, and choroid.18,27
Anterior Segment
Imaging of the anterior segment with OCT allows visualization of the cornea, sclera, limbus, iris, and front surface of the lens.9 The tissues with the highest backreflectance and backscattering intensity, which appear bright white on anterior segment OCT (ASOCT), are the sclera and the tear film. The corneal epithelium and endothelium have higher backreflectance and backscattering than the intervening stroma and appear brighter (Fig. 42-3). The Visante OCT system and SL-OCT are two commercially available slitlamp mounted anterior segment TD-OCT systems. They both provide automatic calculation of biometric parameters such as anterior chamber diameter, anterior chamber depth, corneal curvature, corneal thickness, anterior chamber angle dimensions, and lens vault. Built-in algorithms can also calculate laser in situ keratomileusis (LASIK) flap thickness and residual stromal bed thickness postoperatively. The use of an integrated optometer allows measurement of changes in anterior chamber biometric parameters during accommodation. Prototype anterior segment SD-OCT systems are able to visualize Schlemm’s canal, the trabecular meshwork, and part of the ciliary body.
DETECTABLE ABNORMALITIES
Pathologic changes in the retina that are detectable by OCT appear as either morphologic alterations of the retinal contour or as alterations of backscattering properties of the retinal layers. Pathologic conditions that are detectable as alterations in retinal contour and morphology include macular edema, macular hole, pseudohole, lamellar hole, epiretinal membrane, vitreous detachment, neurosensory retinal detachment, RPE detachment, retinoschisis, retinal dystrophies, and central serous chorioretinopathy.28 OCT allows discrimination between macular hole, pseudohole, and lamellar hole with a high degree of accuracy and can be used to follow the progression of macular holes.29 Retinal detachment, retinoschisis, and RPE detachment can also be reliably discriminated from one another.30
Retinal inflammation, hemorrhage, fibrosis, hard exudates, and vascular proliferation are detectable as alterations in backscattering properties.28 Blood vessels, hemorrhages, and hard exudates are highly backscattering and produce shadowing of the underlying tissue. Serous fluid, on the other hand, is optically transparent and appears weakly backscattering. Subretinal exudate has a backscattering intensity that is an intermediate between that of blood and serous fluid.
A particularly informative feature of OCT is its ability to quantify abnormalities. In the case of macular edema, for example, OCT can be used not only for detection, but also for quantitative monitoring of disease progression and effectiveness of therapy by using serial measurements of foveal thickness.31 Similarly, detection of glaucoma using OCT relies on measurement of the RNFL thickness, which, if monitored over time, can theoretically provide an estimate of disease progression.25 One of the challenges in applying this principle to routine clinical use, however, is the difficulty of establishing representative normative databases for the measurements.32 Another challenge is the poor reproducibility of the measurements of RNFL and macula using the Stratus OCT. SD-OCT systems may overcome this shortcoming owing to data sampling at much higher speed.
The primary utility of OCT in corneal and anterior segment imaging, with few exceptions, is for biometric measurements rather than detection of abnormalities. These include measurement of anterior chamber width for determining the appropriate-sized anterior chamber intraocular lens to implant; sequential measurements of iris nevi and iris masses; measurement of cataract dimensions, density, and capsular proximity; detection of occludable angles; accurate corneal thickness measurements prior to LASIK; and survey of corneal and anterior segment surgical results.33 Diagnostic possibilities of this imaging modality also include the detection of keratoconus.33 The prototypic SD-OCT systems are also able to visualize cyclodialysis clefts34 and iris cysts.22
RNFL THICKNESS ANALYSIS USING OCT
Accurate early diagnosis of glaucoma, particularly pre-perimetric glaucoma (structural damage to the RNFL preceding the onset of visual field loss on standard automated perimetry [SAP]), and detection of disease progression over time are both formidable challenges in managing patients with suspected or known glaucoma. The clinical gold standard in diagnosis and follow-up of progression of glaucomatous optic neuropathy is a combination of stereoscopic ONH examination and visual field testing by SAP. However, the results of ONH examination by ophthalmoscopy and stereoscopic photographs depend on the skill level of the observer, and the reliability of these techniques is undermined by high interobserver and intraobserver variability.35,36 Although substantial advances have been made in developing highly sensitive automated perimetry devices for early glaucoma detection (such as FDT, frequency-doubling technology,37 and SWAP, short-wavelength automated perimetry),38 the utility of these tests requires patient cooperation and performance using subjective measures and can be affected by patient effort. Occasionally, repeated testing is necessary to confirm abnormal results, thereby delaying diagnosis and treatment.39 Moreover, it is widely believed that structural examination of the RNFL for early detection of glaucomatous damage is superior to the aforementioned methods because RNFL damage has often been shown to precede the onset of visual field deficits.40,41
Several imaging technologies have been developed for early detection of RNFL damage, including CSLO (performed by HRT, Heidelberg Retina Tomography, Heidelberg Engineering, Dossenheim, Germany), and scanning laser polarimetry (SLP, performed by GDx, Laser Diagnostic Technologies, Inc., San Diego, CA). HRT uses confocal imaging technology to acquire coronal sections of the ONH and calculate the height of the ONH from a standardized reference plane, which is 50 μm posterior to the average retinal height at the temporal disc margin over a six-degree slice just inferior to the horizontal line bisecting the ONH (350°–356°). It then displays the results as a topographic map of the ONH and calculates multiple parameters that quantify the shape of the ONH, including cup-to-disc ratio and neuroretinal rim area.42 A limitation of this technique is that glaucomatous optic nerve atrophy leads to posterior displacement of the reference plane since the entire retinal thickness at the disc margin consists of the RNFL. This, in turn, alters the results of the topographic parameter calculations that are used to diagnose glaucoma.43 Surprisingly, this effect is even observed in glaucomatous eyes with good visual acuity, where, from physiologic data, it was presumed that the RNFL thickness remains constant until late in disease progression owing to preservation of the papillomacular bundle.43 SLP calculates RNFL thickness as a function of the amount of retardation of polarized light.44 Initially the utility of this technique was limited by the presence of polarizing structures in the anterior segment, which were not individually compensated for in each patient during the RNFL thickness calculation. The new GDx-VCC (variable corneal compensation) instrument (Laser Diagnostic Technologies, Inc.) has addressed this problem by measuring the polarization component contributed by the anterior segment in each patient and removing it from RNFL thickness calculation.45
Unlike the aforementioned methods, the use of OCT for detection of glaucoma is based on direct measurement of RNFL thickness. The most extensively studied protocol is a 3.4-mm-diameter circular scan consisting of 100 A-scans centered around the ONH, referred to as a peripapillary scan.46 This scan produces a cylindrical section of the retina that is then ‘unrolled’ and displayed as a two-dimensional tomograph. The OCT software is able to calculate the RNFL thickness by automated detection of its anterior and posterior boundaries. The anterior and posterior boundaries of the highly reflective RNFL are defined by searching dorsally starting in the vitreous and ventrally starting in the photoreceptor layer, respectively. The scan is typically acquired three times, and the average thickness value at each location is used for further analysis. These data can be used to calculate the RNFL overall mean thickness as well as the average thickness by quadrant (90° each: superior (S), inferior (I), temporal (T), nasal (N)), and by clock-hour (30° each). These RNFL thickness measurements are then compared to a normative database and categorized as either normal, borderline, or abnormal (Figs. 42-4 and 42-5). Abnormally thin measurements are attributable to glaucoma in the absence of potentially confounding historical or funduscopic exam findings such as myopic degeneration. The RNFL thickness measurements can also theoretically be used to follow glaucomatous damage over time. It is important to recognize that for this technology to be successfully used in routine clinical glaucoma practice, it needs to reproducibly measure RNFL thickness and correlate RNFL thinning with functional impairment (i.e., visual field defects). Furthermore, it must demonstrate adequate sensitivity and specificity for identifying patients with glaucoma and determining whether a patient with glaucoma is progressing.
REPRODUCIBILTY AND ACCURACY OF RNFL THICKNESS MEASUREMENTS WITH OCT
Measurement variability can mask the difference between RNFL thickness distributions of normal and glaucomatous populations, thus precluding accurate assignment of individuals to either group based on OCT results. Several sources of variability are inherent in OCT measurements: intersubject variability (normal distribution of RNFL thickness in the population), refractive error, intrasubject variability (owing to eye movement, patient positioning, instrument operator, and other unexplained factors), and instrument noise (owing to sampling density, resolution, and border-finding algorithm).
Two studies assessed intersubject variability by examining the effects of age, optic disc area, race, eye, sex, and axial length on RNFL measurements by Stratus OCT. One study found that RNFL thickness varies significantly with age, ethnicity, axial length, and optic disc area, whereas eye and sex do not have a significant impact.47 Thinner RNFL measurements are seen in whites (p = 0.006), individuals of older age (p <0.001), persons with greater axial length (p <0.001), and those with smaller optic disc area (p = 0.010).47 In a small study, whites had mean RNFL values of 98.1 ± 11.6 μm, which were thinner than those of Asians (105.8 ± 9.2 μm) and blacks (101.1 ± 14.0 μm).32 The current version of the Stratus OCT software adjusts for age but not for other variables such as race, axial length, or optic disc size. Additional studies with larger sample sizes are required to determine whether these and other variables require adjustment.
Regarding intrasubject variability, studies have shown that the mean RNFL values decrease with increasing scan diameter (increasing distance from the optic nerve) in a manner consistent with histologic data.48 Additionally, it is clear that with decreasing distance from the disc margin the RNFL thickness underestimation by OCT becomes significant.49 A plausible explanation for this observation is that the change in orientation of the nerve fibers with respect to the incident OCT light beam as they approach the optic disc, as well as a lack of perpendicularity of the incident OCT beam with the tissue surface, causes a change in measured reflectivity that affects the thickness calculation by OCT software.49 Alternatively, RNFL thickness measurements may be falsely elevated in peripapillary regions, where the ganglion cell layer (GCL) is thin, consisting of two or fewer nuclei (10–20 μm), because the scan resolution is not sufficient to discriminate the GCL from the overlying RNFL.50 In another study, Wu et al51 determined that signal strength variability, which is a measure of the amount of light reflected from the retina, did not impact the measurements as long as it was consistent in serial measurements. They also looked at instrument noise and found that a low analysis confidence, which is a measure of the quality of the data reported by the OCT software, is associated with a lower average RNFL thickness and a greater difference in RNFL thickness between the initial and repeat scans.51
Using OCT-1, Schuman et al46 determined that the optimum scanning protocol based on reproducibility is a 3.4-mm-diameter peripapillary scan consisting of 100 A-scans, using internal fixation with patching of the fellow eye. Carpiento et al52 tested the effect of using different scan radii determined as multiples of the ONH radius (R). The rationale for this approach is that RNFL thickness decreases with increasing distance from the OHN48; therefore, if one uses a standard scan diameter, the scan is closer to the ONH in eyes with large nerves than in eyes with small nerves, leading to artifactually inflated measurements. Scans acquired at a scan radius of 1.5 R showed only slightly improved reproducibility in both normal and glaucomatous eyes compared to the standard 3.4-mm-diameter scans. Mok et al53 showed that increasing the number of averaged scans per session from 3 to 7 led only to modest improvement in reproducibility of quadrant and clock-hour measurements but not in mean RNFL.
Blumenthal et al54 studied the reproducibility of OCT 2000 and demonstrated an improvement over the first published prototype OCT instrument. Coefficients of variation by quadrant were lowest in the inferior and superior quadrants, the areas earliest affected by glaucoma. These investigators also determined the impact of session (measurements on the same day), visit (measurements on different days), and operator on the variability of RNFL measurements to be 1%, 5%, and 2%, respectively. Most of the variance (79%) was a result of interpatient variation, and the remaining 13% of the variance was a result of scan-to-scan noise. Villain and Greenfield55 further demonstrated, using OCT 2000, that even inexperienced operators can achieve RNFL thickness measurements that are adequately reproducible. Reproducibility is generally poorer in glaucomatous eyes.46,54 Reasons why these eyes have reduced measurement reproducibility may be related to inclusion of patients with different stages of disease in the glaucoma group, or from increased difficulty of automated posterior RNFL boundary determination in glaucomatous eyes associated with a decrease in RNFL reflectivity.56
Two studies have compared RNFL thickness measurements obtained with OCT 200057 and Stratus OCT49 to previously published histologic data from human cadaver eyes48,58 and determined that OCT underestimated RNFL thickness by as much as 45% depending on scan diameter and quadrant. Skaf et al,49 using manual RNFL thickness measurement with the caliper tool in the OCT software, obtained thickness values that were not significantly different from the histologic data, indicating that the measurement discrepancy is likely secondary to flawed RNFL boundary-finding algorithms in the software. However, this assessment of accuracy of OCT measurements assumes that the histologic measurements with which the scans are being compared are accurate and can be extrapolated to the OCT subject population. A study by Bourne et al59 compared the RNFL thickness measurements obtained by OCT 2000 and Stratus OCT. In most eyes, RNFL thickness measurements obtained with Stratus OCT were thinner than measurements of the same eyes obtained with OCT 2000. The proprietary nature of OCT algorithms makes it challenging to determine why there are discrepancies among these devices. Another study compared the results of RNFL thickness and fast RNFL thickness protocols with Stratus OCT and demonstrated that the protocols produce different results.60 Until researchers are better able to understand the effect of different testing parameters on RNFL thickness measurements and develop techniques for adjusting for such differences, clinicians may not be able to use serial OCT measurements for the longitudinal monitoring of disease progression. Although differences in measurements between instrument versions may not pose a significant challenge to glaucoma detection if they are systematic and comparable in the normal and glaucoma-suspect populations, longitudinal follow-up of glaucoma progression using OCT may necessitate consistent use of the same instrument version, software version, and scanning protocol.61
With improved imaging speed, the Stratus OCT is capable of acquiring 512 A-scans/circle as the “regular” RNFL thickness scan (1.28 seconds per circle). The “fast” RNFL thickness scan mode consecutively acquires and averages three 256 A-scan circles (0.64 seconds per circle, 1.8 sec. total). In two studies, the increased sampling density of the regular RNFL scan did not offer any improvement in reproducibility over the fast RNFL scan.62,63 Pupillary dilation is recommended by the manufacturer for RNFL thickness measurements using the Stratus OCT, although it has not been shown to be necessary for all patients.64,65,66 It is also recommended that the testing conditions not vary between visits.66
Recently, Mumcuoglu et al67 questioned whether further improving the scanning speed and axial resolution would improve the visualization of glaucomatous structural changes. Using novel scanning patterns from a prototype SD-OCT, which has an axial resolution three times higher than Stratus OCT and an A-scan rate 60 times faster than Stratus OCT, they were able to delineate abnormalities in the GCL and other areas of structural damage attributable to glaucoma.
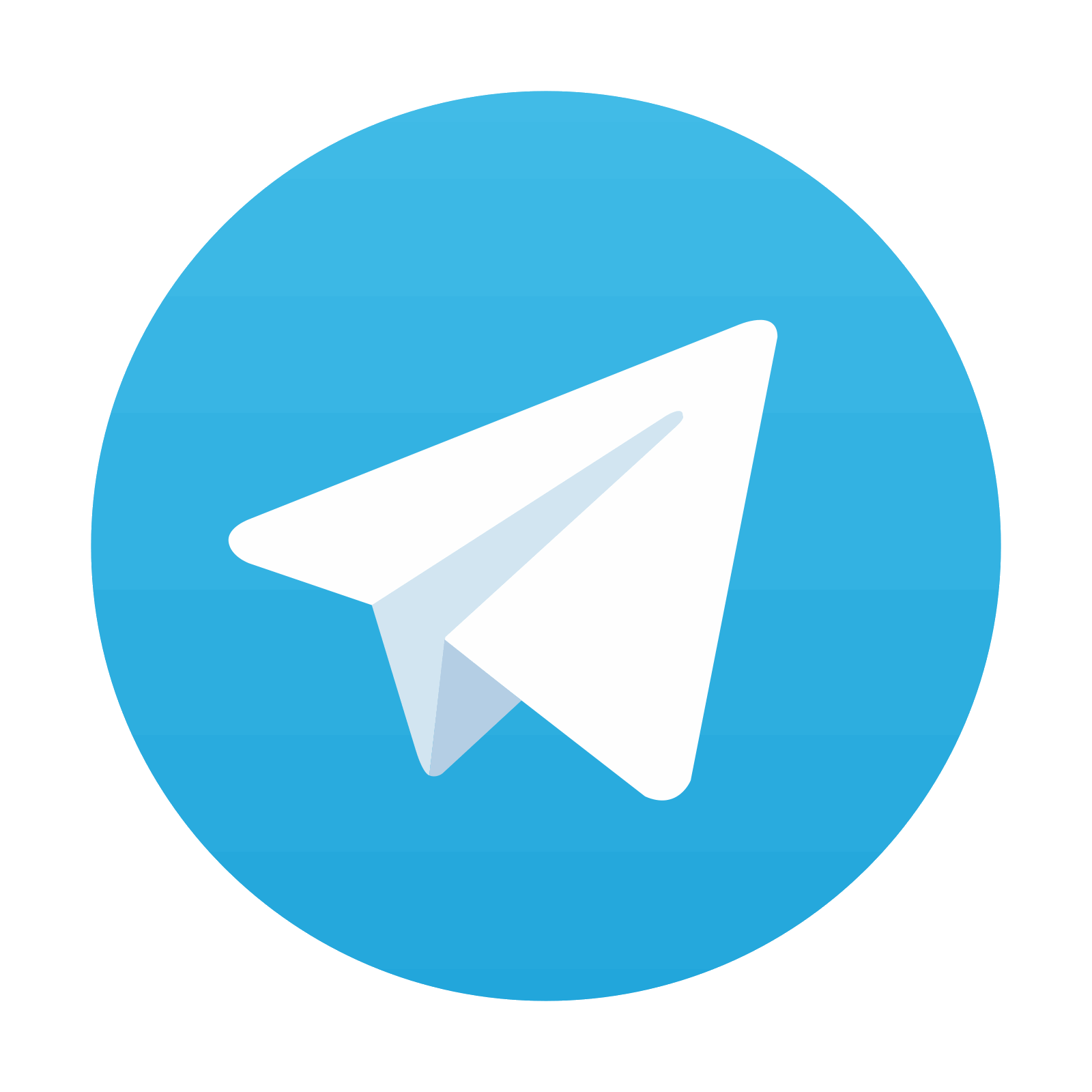
Stay updated, free articles. Join our Telegram channel
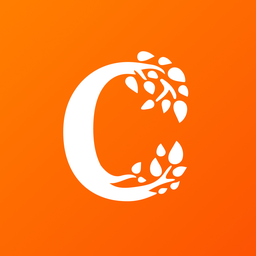
Full access? Get Clinical Tree
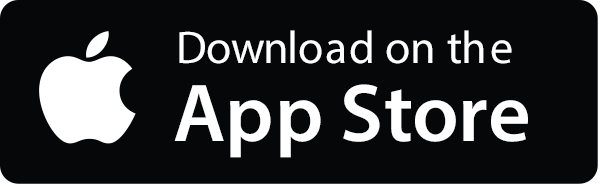
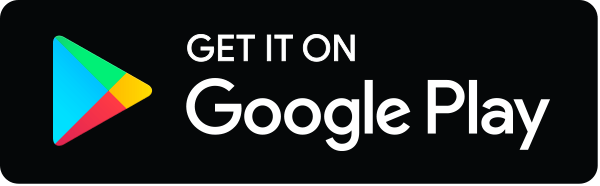