Anatomical terms of reference
The internationally accepted terminology for description of the relations and position of structures in the body requires reference to a series of imaginary planes ( Fig. 1-1 ). Thus, relative positions of anatomical structures are referred to in terms of: medial (nearer the median or mid-sagittal plane) and lateral (away from this plane); anterior and posterior refer to the front and back surfaces of the body; superior (cranial or rostral) or inferior (caudal) refer to position in the vertical; superficial and deep specify distance from the surface of the body. A combination of terms can be used to describe the relative position of structures that do not fit exactly any of the other terms, e.g. ventrolateral, posteromedial, etc.

Osteology of the skull and orbits
General arrangement and features of the skull
The skull is divided into two parts: an upper part shaped like a bowl, which contains the brain, known as the cranium or neurocranium ; and a lower part, the facial skeleton or viscerocranium. The cranium can be further subdivided into the cranial vault and cranial base.
The skull is composed of a large number of separate bones that are united by sutures (fibrous immovable joints). The cranium consists of eight bones (only two are paired), the facial skeleton consists of 14 bones, of which only two are single ( Fig. 1-2A,B ). The skull contains a number of cavities that reflect its multiple functions:
- •
cranial cavity – houses, supports and protects the brain
- •
nasal cavity – concerned with respiration and olfaction
- •
orbits – contain the eyes and adnexa
- •
oral cavity – start of gastrointestinal tract, responsible for mastication and initial food processing; houses taste receptors.



Osteology of the orbit
The orbital cavities, situated between the cranium and facial skeleton, are separated from each other by the nasal cavity and the ethmoidal and sphenoidal air sinuses ( Fig. 1-3A–C ). Each bony orbit accommodates and protects the eye and adnexa, and serves to transmit the nerves and vessels that supply the face around the orbit. Parts of the following bones contribute to the walls of the orbit: maxilla, frontal, sphenoid, zygomatic, palatine, ethmoid and lacrimal ( Figs 1-4 and 1-5A,B ). Each orbit is roughly the shape of a quadrilateral pyramid whose base is the orbital margin and whose apex narrows at the optic canal. Each orbit has a floor, roof, medial wall and lateral wall ( Fig. 1-4 ). (See video 1-2).


The floor tapers off before reaching the apex; therefore the apex of the pyramid is triangular in shape. The orbit is widest approximately 1.5 cm behind the orbital margin. The medial walls are approximately parallel to the mid-sagittal plane, while the lateral walls are oriented at an angle of approximately 45° to this plane. The orbital aperture is directed forwards, laterally and slightly downwards, a characteristic of primates and indeed predators which require binocular vision. Nerves and muscles passing from the apex into the orbit pass forward and laterally ( Fig. 1-3A,B ). The orbit is approximately 40 mm in height, 40 mm in width and 40 mm in depth. The volume is approximately 30 mL, of which one-fifth is occupied by the eye.
The walls of the orbit
The bones that make up the roof, floor and medial and lateral walls are summarized in Figure 1-4 .
Features of the orbital roof
- •
Fossa for the lacrimal gland: lies in the anterolateral aspect of the roof behind the zygomatic process of the frontal bone.
- •
Trochlear fossa (fovea): lies in the anteromedial aspect of the roof, 4 mm from the margin, and is the site at which the trochlea (small pulley) is attached. The tendon of the superior oblique passes through the trochlea.
- •
Anterior and posterior ethmoidal canals Positioned at the junction of roof and medial wall above the frontoethmoidal suture ( Fig. 1-5A ). They transmit the anterior and posterior ethmoidal nerves and vessels.
Relations.
The roof, which is thin and translucent except at the lesser wing of the sphenoid, separates the orbit from the anterior cranial fossa and frontal lobes of the brain. Anteriorly, the frontal sinus lies above the orbit.
Features of the medial orbital wall
- •
This wall is oblong in shape and thin (0.2–0.4 mm). The four bones that comprise this wall are separated by vertical sutures ( Figs 1-4 and 1-5A ).
- •
Lacrimal fossa for the lacrimal sac: it is bound by anterior and posterior lacrimal crests and is continuous below with the nasolacrimal canal ( Fig. 1-5B ).
Relations.
This is the thinnest of the walls and is largely transparent or semitransparent – the ethmoidal air sinuses can easily be seen through this wall in a dried skull ( Fig. 1-5A,B ). Medial to this wall in an anterior to posterior sequence lie the anterior, middle, posterior ethmoidal air cells and the sphenoidal sinus.
This condition may be a consequence of infection spreading from the air sinuses to the orbit via the paper-thin medial wall ( lamina papyracea ) that separates the two. An example is shown of a patient with orbital cellulitis following creation of a drainage fistula ( A ). A coronal CT ( B ) illustrates the communication with the ethmoidal sinuses and nasal cavity (arrow).

Features of the orbital floor
- •
The floor slopes slightly downwards from the medial to the lateral wall.
- •
It is crossed by the infraorbital groove , which runs forward from the inferior orbital fissure. Before it reaches the orbital margin this fissure becomes the infraorbital canal , which opens as the infraorbital foramen 4 mm below the orbital margin on the anterior surface of the maxilla ( Figs 1-3C, 1-4 and 1-5A ).
Relations.
The floor separates the orbit from the maxillary sinus, the bone being only 0.5–1 mm in thickness ( Fig. 1-3C ).
The floor, although thicker than the medial wall, is more often involved in orbital blow-out fractures, probably because it lacks the buttress-like supports of the ethmoidal air cells and the protection of the nose. Tumour spread to or from the maxillary sinus may occur via the floor of the orbit.

An example of ocular motility being compromised in left eye following blow-out fracture ( A ) and a coronal CT ( B ) showing the herniation of orbital contents into the roof of the maxillary sinus (arrow).
Features of the lateral orbital wall ( Fig. 1-4 )
- •
Spina recti lateralis: a small bony spine on the greater wing of the sphenoid near the apex of the orbit which gives origin to part of the lateral rectus.
- •
Zygomatic foramen: transmits zygomatic nerve and vessels to temporal fossa and cheek (zygomaticotemporal nerve and zygomaticofacial nerve) ( Fig. 1-5B ).
- •
Lateral orbital tubercle: forms the attachment of the check ligament of the lateral rectus, suspensory ligament (Lockwood’s) of the eye, superior transverse ligament (Whitnall’s) and aponeurosis of levator palpebrae superioris.
- •
Foramina for small veins that communicate with middle cranial fossa.
Relations.
Laterally – skin, temporal fossa and middle cranial fossa in an anterior–posterior sequence ( Fig. 1-3A ).
Orbital margin, fissures and optic canal
Orbital margin.
This is a thickened rim of bone that helps protect the orbital contents. It is made up of three bones: the frontal, zygomatic and maxilla ( Figs 1-4 and 1-5A,B ). The lateral margin does not reach as far anteriorly as the medial margin (see Figs 1-2B and 1-5B ). The medial margin is sharp and distinct in its lower half because of the anterior lacrimal crest, but is indistinct superiorly ( Figs 1-4 and 1-5B ).
Superior orbital fissure.
This communication between the orbital and cranial cavities lies between the roof and lateral wall of the orbit and is bounded by the lesser and greater wings of sphenoid ( Figs 1-4, 1-5A and 1-6 ). It is wider at its medial end and narrowest at its lateral end. It is around 22 mm long and is separated from the optic foramen above by the posterior root of the lesser wing of the sphenoid. The part of the common tendinous ring that gives origin to the lateral rectus spans between the narrow and wide parts of the fissure. Structures passing above or outside the tendinous ring or annulus include the lacrimal nerve, frontal nerve, trochlear nerve, superior ophthalmic vein and recurrent branch of the lacrimal artery. The latter anastomoses with the orbital branch of the middle meningeal artery and may more commonly travel in a small cranio-orbital foramen lateral to the superior orbital fissure. Structures passing within the ring, and thus within the apex of the muscle cone, include the oculomotor nerve (superior and inferior divisions), abducent nerve, nasociliary nerve, sympathetic root of the ciliary ganglion, and variably the inferior ophthalmic vein ( Fig. 1-6 ).

Inferior orbital fissure.
This fissure lies between the lateral wall and floor of the orbit below the superior orbital fissure. It forms a communication between the orbit and the infratemporal fossa and pterygopalatine fossa. It runs forward and laterally for approximately 20 mm and ends 20 mm from the orbital margin ( Figs 1-4 and 1-5A ). The fissure is narrowest in the middle section and in life is covered by periorbita and a sheet of smooth muscle of unknown function, the orbitalis or ‘muscle of Müller’. It transmits the infraorbital nerve, zygomatic nerve, branches from the pterygopalatine ganglion and the inferior ophthalmic vein may communicate with the pterygoid venous plexus below.
Optic canal.
This is a bony channel in the sphenoid that passes anteriorly, inferiorly and laterally (36°) from the middle cranial fossa to the apex of the orbit. The canal is formed by the two roots of the lesser wing of the sphenoid. The optic canals are 25 mm apart posteriorly and 30 mm anteriorly. Each is funnel-shaped and narrowest anteriorly where its opening into the orbit is oval with sharp upper and lower borders and a prolonged roof (10–12 mm in length). The opening at the cranial aspect is oval with a prolonged floor. The sphenoidal and posterior ethmoidal air sinuses are important medial relations, and the olfactory tracts are superior relations of the canal. The canal transmits the optic nerve with its meningeal coverings and the ophthalmic artery , which lies below and then lateral to the nerve within the dural sheath for part of its course ( Fig. 1-6 ). Sympathetic nerve fibres accompany the artery.
Paranasal sinuses
The paranasal sinuses ( Figs 1-3 , 1-7 and Video 1.3 ) comprise the frontal, ethmoidal, sphenoidal and maxillary sinuses. They are air-filled cavities in the skull that are in communication with the nasal cavity via a series of apertures. Infection commonly spreads from the nasal cavity into the sinuses. The sinuses function to warm and moisten the air, add resonance to the voice and lighten the skull. They vary in size and shape between individuals.



Frontal Sinuses ( Figs 1-4 and 1-7 )
The frontal sinuses are paired and lie behind the superciliary arches within the frontal bone ( eFig. 1-1 ). They are separated from each other or further subdivided by thin bony septa that are not necessarily in the midline. The sinuses may extend as far laterally as the zygomatic process of the frontal bone. Each is approximately triangular and extends highest above the medial end of the eyebrow ( Fig. 1-7 ). Each sinus opens into the middle meatus of the nasal cavity, either through the ethmoidal infundibulum or directly via the frontonasal duct. The mucosal lining is supplied by the supraorbital nerves and vessels; hence, referred pain from frontal sinusitis is experienced along the course of the supraorbital nerve.

Recent geometric morphometric studies (elliptic Fourier analysis) of the outlines of frontal sinuses from large numbers of radiographic images have confirmed a long-held belief that each individual’s frontal sinus is distinct and unique. This may have important applications for personal identification in the context of forensics.
Ethmoidal Sinuses (Air Cells) ( Figs 1-3A–C and 1-7 )
These thin-walled sinuses are for the most part situated in the lateral mass of the ethmoid, although frontal, maxillary, lacrimal, sphenoidal and palatine bones contribute to the walls. They are variable in number and are grouped into anterior, middle and posterior. The general pattern of drainage of the sinuses is as follows: the anterior opens into the hiatus semilunaris, the middle on to the bulla ethmoidalis (both middle meatus) and the posterior into the superior meatus. They are related to the frontal sinus anteriorly, the sphenoidal sinus posteriorly, the nasal cavity medially and below, and laterally the orbit ( Fig. 1-7A ).
Sphenoidal Sinus ( Figs 1-3A,B and 1-7 )
This sinus lies within the body of the sphenoid bone and possesses an indented roof because of the pituitary fossa that lies above and houses the pituitary gland ( Fig. 1-8 ). It may be divided by a variable midline septum. A transverse ridge in the lateral wall marks the position of the internal carotid artery (within the cavernous sinus). Other important relations of the sinus include the optic chiasma and nerves above, the nasal cavity below, the ethmoidal sinuses anteriorly, and the paired cavernous sinuses laterally ( Fig. 1-9C ). The sphenoidal sinus drains into the superior meatus or sphenoethmoidal recess. Surgical access to the pituitary gland may be gained via the nasal cavity and sphenoidal sinus; hence, surgeons must be aware of the above-mentioned relations.




Maxillary Sinus ( Figs 1-3C and 1-7 )
These are the largest of the paranasal air sinuses. They are pyramidal in shape and lie within the body of the maxilla. The base forms part of the lateral wall of the nasal cavity and the apex is within the zygomatic process. Each sinus is in communication with the middle meatus of the nasal cavity via an aperture, the maxillary hiatus, on its base, which empties into the lower part of the hiatus semilunaris. The opening is positioned high on this wall and therefore does not facilitate gravitational drainage in the upright position. The nasolacrimal duct lies in a thin bony canal in the anterior part of the base. The orbital plate forms the roof of the sinus and floor of the orbit.
Orbital Floor Fractures
Rapid traumatic compression of the orbital contents, such as occurs during squash ball injuries, can lead to blow-out fractures; orbital contents may herniate into the maxillary sinus. It was once thought that orbital contents, including extraocular muscles, became trapped in the fractured floor, thus restricting range of movement and explaining the diplopia suffered by these patients. However, recent studies have indicated that in many cases only orbital fibroadipose tissue is trapped in the damaged floor of the orbit (see p. 6 ).
The floor of the maxillary sinus is formed by the alveolar process housing a variable number of the roots of the first and second molars that protrude into the sinus, and may be separated from the sinus by only a thin covering of bone or mucous membrane. Thus sinusitis may present as referred pain such as toothache and vice versa. In addition, abscesses in the maxillary sinus may result from infection of these roots. The anterior/lateral wall is directed on to the face, and access for drainage of maxillary obstructions or other surgical procedures in the sinus may be gained by this route. The posterior wall faces the infratemporal fossa.
Cranial cavity ( Fig. 1-8A,B and eFig. 1-1
)
The cranial cavity houses the brain, cerebral vessels, the meninges, meningeal vessels and the intracranial portions of the cranial nerves. The base of the cranial cavity can be subdivided for descriptive purposes into three fossae: anterior, middle and posterior. Accounts of the detailed anatomy of these fossae can be found in any standard anatomy text; therefore only features of relevance to the eye and orbit in the anterior and middle cranial fossae will be described.
Posterior Cranial Fossa
This is the deepest of the three cranial fossae, its floor lying below the level of the middle fossa. Its roof is formed by the tentorium cerebelli. It lodges the hindbrain: the cerebellum, pons and medulla oblongata. The fossa is bound anteriorly by the superior border of the petrous temporal bone and the dorsum sella, and surrounds the foramen magnum, the cerebellum being housed in the cerebellar fossae on the squamous part of the occipital bone. Features and openings on the floor of the posterior cranial fossa are not as relevant to the eye and orbit as those in the anterior or middle fossae; however, readers should be able to identify the following: foramen magnum, jugular foramen, hypoglossal canal, internal acoustic meatus, grooves for the sigmoid and transverse sinuses, internal occipital protuberance and clivus ( Fig. 1-8A,B ).
Cranial fossae
Anterior cranial fossa.
The anterior cranial fossa is limited in front and laterally by the frontal bone and posteriorly by the lesser wing of the sphenoid. Its floor is formed by the orbital plate of the frontal bone, the cribriform plate of the ethmoid (with a median crest-like ridge, the crista galli, which forms the anterior attachment of the falx cerebri), and the lesser wings and anterior part of the body (jugum) of the sphenoid. The perforations of the cribriform plate transmit the olfactory nerves. The orbital plate of the frontal bone separates the orbit below from the frontal lobes of the cerebral hemispheres, whose sulci and gyri cause surface impressions on the bone. Projecting posteriorly from the lesser wings of the sphenoid are the anterior clinoid processes that overhang the middle cranial fossa and give attachment to the free edge of the tentorium cerebelli.
Middle cranial fossa.
The middle cranial fossa lies at a lower plane than the anterior cranial fossa but is higher than the posterior cranial fossa. Its floor is shaped like a butterfly, with a narrow central or median part and expanded lateral parts. It is bound anteriorly by the posterior free edge of the lesser wing of the sphenoid, the anterior clinoid processes, and the anterior margin of the sulcus chiasmaticus ( Fig. 1-8A,B ). Posteriorly it extends to the superior borders of the petrous temporal bones and dorsum sellae of the sphenoid, and laterally it is bound by the squamous part of the temporal bone, part of the parietal bones, and the greater wings of the sphenoid. Features and foramina of the floor of the middle cranial fossa and the structures that they transmit are summarized in Table 1-1 .
Feature/foramen | Position | Relevance |
---|---|---|
Sulcus chiasmaticus | Between the two optic canals anterior to tuberculum sella | Only rarely does optic chiasma lie in contact with this region |
Sella turcica (‘Turkish saddle’) | Central part of sphenoid body between the two cavernous sinuses | The central hollow, the hypophyseal fossa, houses the pituitary gland. Anterior and posterior clinoid processes give attachment to the free and attached margins of the tentorium cerebelli |
Optic canal | Between the two roots of the lesser wing of the sphenoid | Transmits optic nerve, ophthalmic artery, sympathetic nerves and meningeal coverings |
Superior orbital fissure | Between the lesser and greater wings of the sphenoid. Lies at apex of cavernous sinus | Transmits trochlear, abducent and oculomotor nerves and terminal branches of ophthalmic nerve |
Foramen rotundum | Pierces greater wing of sphenoid | Transmits maxillary nerve and small veins from cavernous sinus |
Foramen ovale | Pierces greater wing of sphenoid | Transmits mandibular nerve, accessory meningeal artery and occasionally the lesser petrosal nerve |
Foramen spinosum | Posterolateral to foramen ovale | Transmits middle meningeal artery and vein and meningeal branch of the mandibular nerve |
Foramen lacerum | At apex of petrous temporal bone | The upper end transmits the internal carotid artery before it enters the cavernous sinus. Also transmits sympathetic nerves and a small plexus of veins. The lower end is covered by connective tissue and pierced only by small branches of the ascending pharyngeal artery |
Trigeminal impression | Anterior surface of petrous temporal bone behind foramen lacerum | Occupied by trigeminal ganglion in trigeminal cave. Joined on lateral aspect by grooves for the greater and lesser petrosal nerves |
Tegmen tympani and arcuate eminence | Tegmen is a thin plate of temporal bone over middle ear cavity. Arcuate eminence is produced by superior semicircular canal in petrous temporal bone | Infections in middle ear may spread through thin plate of bone to middle cranial fossa and temporal lobe of the brain |
Pituitary fossa.
The pituitary fossa (hypophyseal fossa) is an indentation in the roof of the body of the sphenoid bone in the middle cranial fossa. It is bound anteriorly by the tuberculum sellae , in front of which lies the sulcus chiasmatica , and posteriorly by the dorsum sellae , a ridge of bone at either end of which lies the posterior clinoid processes. The pituitary fossa houses the pituitary gland or hypophysis cerebri. This is connected by a thin stalk – the pituitary stalk (or tuber cinereum) – to the brain. The fossa is roofed by a sheet of dura mater, the diaphragma sella ( Fig. 1-9C,D ) which is attached in front to the tuberculum and behind to the dorsum sellae. The pituitary stalk passes through a small opening in the roof. The right and left cavernous sinuses are important lateral relations ( Fig. 1-9C ).
The meninges ( Fig. 1-9A,B and eFig. 1-2A
)


The brain and spinal cord are surrounded by three layers of meninges: a tough pachymeninx , the dura mater, and the leptomeninges consisting of the arachnoid mater and pia mater. Between the arachnoid and pia is the subarachnoid space filled with cerebrospinal fluid.
Dura mater.
The dura mater is theoretically ‘divided’ into an endosteal layer (really the periosteum on the inner surface of the skull) and a meningeal layer; however, on the whole they are fused except where they separate to form dural venous sinuses and dural folds ( Fig. 1-9A,B ). The latter are connective tissue septae that extend into the cranial cavity and serve to subdivide it into compartments. In association with the cerebrospinal fluid they aid in providing physical support and protection for the brain. The position and form of the dural folds are summarized diagrammatically in Figure 1-9A .
The dural venous sinuses are valveless, highly specialized, firm-walled veins within the cranial cavity which drain venous blood from the brain and cranial bones ( Fig. 1-9B ). In common with other veins the sinuses are lined by vascular endothelial cells; however, their walls contain no smooth muscle cells. The arrangement of the sinuses is summarized in Fig. 1-9A and eFig. 1-2B .
Of particular note to those studying the eye and orbit is the pair of cavernous sinuses lying either side of the body of the sphenoid ( Fig. 1-9C,D ).
The importance of the cavernous sinuses ( Fig. 1-9C,D ) lies in their position, relations and extensive communications. Each cavernous sinus is around 2–3 cm long in the sagittal plane and consists of a series of incompletely fused venous channels or a single venous channel partially subdivided by trabeculae. It has walls of dura mater, like other venous sinuses.
Position.
There is one cavernous sinus on either side of the body of the sphenoid. The sinus extends from the superior orbital fissure in front to the apex of the petrous temporal bone behind.
Relations.
These are summarized in Figure 1-9C and D (coronal section).
Communications.
The sinuses communicate with each other via the anterior and posterior intercavernous sinuses. Tributaries draining into the sinuses anteriorly include the superior and inferior ophthalmic veins (which drain the eye and orbit as well as areas of skin around the periorbital region of the face and nose), and the sphenoparietal sinuses. The superficial cerebral vein from the brain drains into the sinus from above ( eFig. 1-2B ).
Blood from each sinus may, depending on relative pressures, drain via the superior and inferior petrosal sinuses either directly to the internal jugular veins (inferior petrosal) or to the transverse sinuses and thus to the internal jugular veins. Other exits include venous plexi around the internal carotid artery or veins traversing the foramen ovale or sphenoidal emissary foramen to communicate with the pterygoid plexus and other veins in the region of the skull base. Communications with the vertebral venous plexus in the epidural space also exist via the basilar venous plexus on the clivus.
Arteriovenous Fistulas in the Cavernous Sinus
These cause a variety of symptoms including pulsating protrusion of the globe and congestion of the vessels of the lids and conjunctiva owing to raised venous pressure. Patients complain of hearing noises resembling rushing water, probably because of increased flow rates in the labyrinthine plexus, which is in communication with the cavernous sinus via the superior petrosal sinus.
Cavernous Sinus Thrombosis
Cavernous sinus thrombosis as a sequel to infection spreading to the sinus, from such diverse initial sites as the nose, lids, behind the ear, bony labyrinth, pharynx and temporomandibular joint, can give rise to a variety of symptoms explainable on the basis of structures affected in and around the sinus. Facial pain may be the result of the involvement of the ophthalmic nerve (V 1 ). Lateral rectus paralysis may follow involvement of the abducent nerve. Involvement of the other oculomotor nerves is less common because they are more protected in the lateral wall of the sinus. Thrombosis is usually bilateral because of the communications via the intercavernous sinuses. Papilloedema may result from obstruction of central retinal venous return.
Falx Cerebri
A sickle-shaped fold with its attached border in the mid-sagittal plane from the crista galli to the tentorium cerebelli behind. It lies in the vertical fissure between the two cerebral hemispheres, its lower border lying above the corpus callosum. The superior sagittal sinus is situated in the attached border and the inferior sagittal sinus is in the lower free border of the falx cerebri.
Tentorium Cerebelli. This fold lies approximately in a horizontal plane at 90° to the falx, although it is elevated centrally (hence ‘tent-like’). It separates the occipital lobe of each cerebral hemisphere above from the cerebellum in the posterior cranial fossa below. The free edge forms the boundary of the tentorial notch, which separates the forebrain from the hindbrain and ‘houses’ the midbrain. Sinuses related to the tentorium include the straight sinus, right and left transverse sinuses, superior petrosal sinuses and cavernous sinuses.
Cavum Trigeminale. A blind-ended dural recess whose entrance is in the posterior cranial fossa. It is formed by an invagination of the dura beneath the free edge of the tentorium and is roofed by dura on the floor of the middle cranial fossa. It houses the trigeminal ganglion, which sits in a shallow hollow on the apex of the petrous temporal bone, and some accompanying vessels. The ganglion is surrounded by cerebrospinal fluid continuous with the subarachnoid space of the posterior cranial fossa.
Diaphragma Sella. A small circular fold of dura over the sella turcica that is pierced centrally by the infundibulum ( Fig. 1-9C ). It blends laterally with the roof of the cavernous sinus ( Fig. 1-9D ).
The area above the tentorium is known as the supratentorial compartment; that below is the infratentorial compartment. The cranial dura of the supratentorial compartment is innervated by sensory branches of the trigeminal nerve, and stimulation of these nerves (stretching, inflammation, compression) gives rise to frontal or parietal headache. The infratentorial compartment is supplied by branches of the upper cervical nerves, and stimulation of these sensory nerves may therefore manifest as occipital and neck pain.
The neck rigidity accompanying acute meningitis of the infratentorial region is most likely the result of reflex contractions, or spasm, of posterior neck musculature in response to stretching of the inflamed cranial and spinal cord meninges.
The meningeal arteries lie within the inner (or periosteal) layer of dura with their accompanying veins ( Fig. 1-9B ) and are responsible for the many fine grooves that ramify over the inner surface of the cranium (see Fig. 1-8B and eFig. 1-1 ).
The largest and most important of these is the middle meningeal artery, which enters the skull through the foramen spinosum. These arteries supply the meninges and diploë (bone marrow of cranial bones), but they do not supply the brain.
Damage to middle meningeal vessels, especially the frontal branch of the middle meningeal artery and vein (the latter lying closest to the bone), may result from blows to the head, especially in the temporal region (the pterion; see Figs 1-2B, 1-5B, 1-8B ) where the bones are thinnest and most likely to fracture. Slow venous, or more rapid arterial, bleeding will lead to an extradural (epidural) haematoma with a resultant rise in intracranial pressure. Coma and death will occur if such a haematoma is not drained as soon as possible after symptoms of raised intracranial pressure, including papilloedema, manifest. A subdural haematoma may occur if the trauma results in brain laceration or tearing of intradural veins.
Arachnoid mater ( eFig. 1-2A
).
The arachnoid ( Gk . spider) is a delicate fibrocellular layer beneath the dura (separated by potential subdural space) that is connected to the pia mater covering the brain by numerous fibrocellular bands that cross the cerebrospinal fluid-filled subarachnoid space. This arrangement has led some to consider the leptomeninges as a conjoined pia–arachnoid membrane. The arachnoid bridges over the sulci, gyri and other irregularities on the brain surface, thus creating the subarachnoid cisterns or enlargements in the subarachnoid space ( Fig. 1-9B , inset, and eFig. 1-2A ).
Specialized regions of arachnoid, the arachnoid villi and granulations (fibrous aggregations of villi), project into several of the dural venous sinuses ( Fig. 1-9B ) and act as one-way pressure-sensitive valves allowing cerebrospinal fluid to drain from the subarachnoid space into the dural venous sinuses. Structures passing to and from the brain to the skull or its foramina, such as cranial nerves, must traverse the subarachnoid space. In addition, all cerebral arteries and veins lie in this space ( eFig. 1-2A ).
Since the arachnoid fuses with the perineurium of cranial nerves, the cerebrospinal fluid-containing subarachnoid space extends for a short distance around all cranial nerves. In particular it surrounds the optic nerve in a cuff-like manner as far as the posterior surface of the eye.
Pia mater ( Fig. 1-9B and eFig. 1-2A
).
The pia mater, a vascular fibrocellular membrane that is thicker than the arachnoid, closely follows the contours of the brain. Vessels entering or leaving the brain parenchyma carry a pial sheath with them. Pial tissue is rich in astrocytes, which extend along the vessel walls and form an important component of the blood–brain barrier. The perivascular spaces surrounding these vessels, the so-called Virchow–Robin spaces, are potentially in communication with the cerebrospinal fluid of the subarachnoid space and may be dilated in pathological states.
Structure of the eye
The eye ( Fig. 1-10 ) is a highly specialized organ of photoreception, the process by which light energy from the environment produces changes in specialized nerve cells in the retina, the rods and cones. These changes result in nerve action potentials, which are subsequently relayed to the optic nerve and then to the brain, where the information is processed and consciously appreciated as vision. All the other structures in the eye are secondary to this basic physiological process, although they may be part of the system necessary for focusing and transmitting the light on to the retina, for example cornea, lens, iris and ciliary body, or they may be necessary for nourishing and supporting the tissues of the eye, for example the choroid, aqueous outflow system and lacrimal apparatus.

General shape, size and position of the eye
The eye is approximately a sphere 2.5 cm in diameter with a volume of 6.5 mL. However, in reality it is the parts of two spheres, a smaller one anteriorly, the cornea, that has a greater curvature than the sclera, which constitutes the large sphere. The cornea forms one-sixth of the circumference of the globe and has a radius of 7.8 mm; the remaining five-sixths is formed by the sclera, which has a radius of 11.5 mm. There is variation in size between individuals but the average axial length of the globe is 24 mm (range 21–26 mm). The diameter is 23 mm and the horizontal length approximately 23.5 mm. Small eyes (<20 mm) are hyperopic or hypermetropic, while large eyes (26–29 mm) are myopic. The eye is situated in the anterior portion of the orbit, closer to the lateral than the medial wall and nearer the roof than the floor. The eye is made up of three basic layers or coats, often known as tunics ( Fig. 1-10 ). These are the fibrous (corneoscleral) coat, the uvea or uveal tract (composed of choroid, ciliary body and iris), and the neural layer (retina). The coats surround the contents, namely the lens and the transparent media (aqueous humour and vitreous body).
The cornea and sclera together form a tough fibrous envelope that protects the ocular tissues. The fibrous coat also provides important structural support for intraocular contents and for attachment of extraocular muscles. The cornea meets the sclera at a region known as the limbus or corneoscleral junction.
The cornea
The surface of the cornea (air–tissue interface) and associated tear film is responsible for most of the refraction of the eye. The transparency of the cornea is its most important property, although because of its highly exposed position it must also present a tough physical barrier to trauma and infection. Corneal transparency is the result of a number of related factors: its avascularity; the regularity and smoothness of the covering epithelium; and the regular arrangement of the extracellular and cellular components in the stroma, which is dependent on the state of hydration, metabolism and nutrition of the stromal elements.
Shape
The cornea is smaller in the vertical (10.6 mm) than in the horizontal (11.7 mm) diameter; however, viewed from behind, the circumference appears circular. The central radius is 7.8 mm with the peripheral corneal curvature being less marked. The cornea is also thicker at the periphery (0.67 mm) than in the centre (0.52 mm).
Astigmatism
Astigmatism is usually the result of differences in the radius of curvature in the vertical and horizontal meridians. Abnormalities in corneal curvature can be readily demonstrated by computerized video keratography, which applies the principle of projecting placido rings onto the corneal surface from which topographic maps can be constructed ( Fig. 1-11 ).

Structure
The cornea is composed of five layers ( Fig. 1-12A ).

Corneal epithelium ( Fig. 1-12B ).
The corneal epithelium is a stratified (possessing five or six layers) squamous non-keratinized epithelium (the superficial cells are flattened, nucleated and non-keratinized). It is 50–60 µm in thickness and adjacent cells are held together by numerous desmosomes and to the underlying basal lamina by hemidesmosomes and anchoring filaments ( Fig. 1-12B ). The anterior surface of the corneal epithelium is characterized by numerous microvilli and microplicae (ridges) whose glycocalyx coat interacts with, and helps stabilize, the precorneal tear film. New cells are derived from mitotic activity in the limbal basal cell layer (see p. 211 ) and these displace existing cells both superficially and centripetally. The corneal epithelium responds rapidly to repair disruptions in its integrity by amoeboid sliding movements of cells on the wound margin followed by cell replication.
The basal epithelial cells rest on a thin, but prominent, basal lamina (lamina lucida, 25 nm; lamina densa, 50 nm). Corneal epithelial adhesion is maintained by a basement membrane complex, which anchors the epithelium to Bowman’s layer via a complex mesh of anchoring fibrils (type VII collagen) and anchoring plaques (type VI collagen), which interact with the lamina densa and the collagen fibrils of Bowman’s layer. The corneal epithelium is devoid of melanocytes. Myeloid-derived major histocompatibility complex (MHC) class II antigen-positive dendritic cells (Langerhans cells) are present in the limbus and peripheral cornea ( Fig. 1-12 ), but decline sharply in density in a centripetal gradient, and are rare in the central cornea. However, MHC class II-negative dendritic cells have been identified in the mouse central cornea and recent in vivo confocal microscopy (IVCM) ( Fig. 1-13 ) suggests that the normal human central corneal epithelium contains dendritic cells although their immunophenotype cannot be ascertained from IVCM. The comparative paucity of potential antigen-presenting cells, such as dendritic cells, and the avascular nature of the cornea are considered factors crucial to the success of corneal grafting (see Ch. 7 ).

Anterior limiting lamina (Bowman’s layer).
Bowman’s layer (a modified acellular region of the stroma; 8–12 µm thick) consists of fine, randomly arranged, collagen fibrils (20–30 nm diameter, types I, III, V and VI). The anterior surface is well delineated and is separated from the epithelium by the thin basal lamina, while the posterior boundary merges with the stroma ( Fig. 1-12A ). Bowman’s layer terminates abruptly at the limbus.
Substantia propria or corneal stroma ( Fig. 1-14 A–C ).
The corneal stroma is a dense connective tissue of remarkable regularity. It makes up the vast majority of the cornea and consists predominantly of 2 µm thick, flattened, collagenous lamellae (200–250 layers) oriented parallel to the corneal surface and continuous with the sclera at the limbus. Between the lamellae lie extremely flattened, modified fibroblasts known as keratocytes. These cells are stellate in shape with thin cytoplasmic extensions containing conspicuously few distinctive organelles ( Fig. 1-14A–C ) when viewed in conventional cross-sections. However, frontal sections reveal an abundance of organelles and a novel network of fenestrations on their surface which may facilitate the diffusion of metabolites or the mechanical ‘anchoring’ or attachment of collagen bundles ( Fig. 1-14A ). The density of keratoctyes in the anterior stroma is 20 000–24 000 cells/mm 2 and that density decreases posteriorly before increasing again near Desçemet’s membrane ( Fig. 1-13C ). Keratocytes are connected by gap junctions to their neighbouring cells and arranged in a corkscrew pattern spiralling from the epithelium to the endothelium. The collagenous lamellae form a highly organized orthogonal ply, adjacent lamellae being oriented at right angles, with the exception of the anterior third in which the lamellae display a more oblique orientation. The collagen fibres ( Fig. 1-14C , inset) are predominantly of type I (30 nm diameter, 64–70 nm banding) with some type III, V and VI also present. The transparency of the cornea is highly dependent on the regular diameter (influenced by the presence of type V collagen in particular) and spacing of the collagen fibres (interfibrillary distance), which in turn is regulated by glycosaminoglycans (GAG) and proteoglycans forming bridges between the collagen fibrils. The GAGs in the human cornea are predominantly keratan sulphate and chondroitin (dermatan) sulphates (see Ch. 4 ). The corneal stroma normally contains no blood or lymphatic vessels, but sensory nerve fibres are present in the anterior layers en route to the epithelium (see below and Fig 1.13 ). Studies using transgenic mice in which eGFP (enhanced green fluorescent protein) is expressed on all CX 3 CR1 positive monocyte derived cells has revealed extensive populations of resident tissue macrophages throughout the corneal stroma, some of which have recently described membrane nanotube cell–cell communications ( Fig. 1-15 ).


Posterior limiting lamina (Desçemet’s membrane) ( Figs 1-12A and 1-16A ).
This is a thin, homogeneous, discrete, periodic acid–Schiff-positive layer between the posterior stroma and the endothelium, from which it can become detached. It is 8–12 µm in thickness and represents the modified basement membrane of the corneal endothelium. It consists of two parts, an anterior third that is banded and a homogeneous or non-banded posterior two-thirds. It is rich in basement membrane glycoproteins, laminin and type IV collagen. The anterior banded region is reported to contain type VIII collagen. Types V and VI collagen may be involved in maintaining adherence at the interface of Desçemet’s membrane with the most posterior lamellae of the stroma. Desçemet’s membrane is continuous peripherally with the cortical zone of the trabeculae in the trabecular meshwork. Microscopic wart-like protuberances (Hassall–Henle bodies) containing ‘long banded’ (100 nm) deposits of unknown nature appear in the periphery of Desçemet’s membrane with age. It is frequently thickened at its peripheral termination (Schwalbe’s line, the anterior limit of the trabecular meshwork). If disrupted, Desçemet’s membrane tends to curl inwards towards the anterior chamber.

Corneal endothelium.
The corneal endothelium, a simple squamous epithelium on the posterior surface of the cornea, has a critical role in maintaining corneal hydration and thus transparency.
There are approximately 350 000 endothelial cells per cornea (3000–4000 cells/mm 2 at birth, falling to 2500 cells/mm 2 in middle age and 2000 cells/mm 2 in old age). Consequently, with age, the dense, regular hexagonal arrangement typical of the young cornea is replaced by fewer cells of more heterogeneous size and shape.
Fluid is constantly being lost via evaporation at the ocular surface, a fact illustrated by increased corneal thickness after a night of lid closure and when an impermeable lens is placed over the epithelium. The endothelial cells rest on Desçemet’s membrane ( Fig. 1-16A ) and form an uninterrupted polygonal or hexagonal array, or mosaic ( Fig. 1-16B ), which can be clearly seen in vivo with the aid of specular microscopy and in vivo confocal microscopy ( Fig. 1-13 ). The cells are 5–6 µm in height and 18–20 µm in diameter. Their lateral surfaces are highly interdigitated and possess apical junctional complexes that, together with abundant cytoplasmic organelles including mitochondria ( Fig. 1-16A ), are indicative of their crucial role in active fluid transport.
In the normal human cornea endothelial cells are generally considered to have low regenerative capacity and lost cells are quickly replaced by spreading of adjacent cells. However, there is some evidence that putative endothelial stem cells are located in centripetally arranged ‘niches’ or columns in the extreme peripheral cornea. Damage to corneal endothelial cells and density below 800 cells/mm 2 leads rapidly to oedema and swelling of the stroma, with resultant loss of transparency (see Ch. 4 , p. 213 ). A density lower than 1500 cells/mm 2 in a potential donor cornea is considered unsuitable for transplantation.
Nerve supply of the cornea
The cornea is richly supplied by sensory fibres derived from the ophthalmic division of the trigeminal nerve , mainly via the long ciliary nerves. Occasionally the inferior cornea receives some branches from the maxillary division of the trigeminal. Nerve bundles enter the peripheral cornea in a radial manner and as they travel centrally below the anterior one-third of the stroma and approximately 1 mm from the limbus they lose their perineurium and myelin sheaths. This alteration in myelination is thought to be related to the importance for transparency. They divide into smaller branches and begin to change direction towards the epithelium where they must pierce Bowman’s layer, whereupon they further divide into smaller bundles to form the subepithelial or sub-basal plexus in the interface between Bowman’s layer and the basal aspect of the corneal epithelium. There are apparently no specialized end organs associated with these terminal axons, which are predominantly within the size range 0.1–0.5 µm consistent with A-delta and C fibres that function to transmit the sensory modalities of pain and temperature. Individual beaded fibres penetrate the epithelial layers and terminate in the superficial layers in the form of an intraepithelial plexus. There are approximately 7000 nociceptors per mm 2 in the human corneal epithelium.
Larger myelinated nerve fibres can often be seen during slit-lamp examination as fine whitish fibres radiating into the cornea from the limbus. In vivo confocal microscopy has greatly added to our understanding of the distribution of corneal nerves in healthy and diseased corneas (see Fig. 1-13 ). Due to their position in the anterior stroma and their radial arrangement, many are damaged during refractive procedures such as LASIK (Excimer laser in situ keratomileusis). Damage to the corneal epithelium and intraepithelial nerve terminals can cause a great deal of pain.
Reactivation of latent herpes simplex virus in the trigeminal ganglion occurs following damage to nerve terminals (cold, exposure to ultraviolet light, trauma, corticosteroids), and activated virus is transmitted to the cornea along sensory nerve branches, leading to recurrent herpes simplex keratitis and superficial corneal ulceration.
The sclera
The sclera ( Fig. 1-17A-D ) forms the principal part of the outer fibrous coat of the eye and functions both to protect the intraocular contents and to maintain the shape of the globe when distended by intrinsic intraocular pressure. The globe shape is maintained even during contraction of the extraocular muscles, whose tendons insert on its surface. The sclera is relatively avascular and in adults appears white externally. The viscoelastic nature of the sclera (great tensile strength, extensibility and flexibility) allows only limited distension and contraction to accommodate minor variations in intraocular pressure.
The corneoscleral envelope of children with congenital glaucoma responds to raised intraocular pressure by irreversibly stretching, owing to the immaturity of the collagen fibres, thus producing the characteristically enlarged buphthalmos (‘ox-eye’) of this condition.

The sclera is thickest posteriorly (1 mm) and thinnest (0.3–0.4 mm) behind the insertions of the aponeurotic tendons of the extraocular muscles. It is covered by the fascia bulbi posteriorly and the conjunctiva anteriorly. The sclera consists of dense irregular connective tissue comprising extracellular matrix and matrix-secreting fibroblasts. The matrix consists principally of collagen type I, although types III, IV, V, VI, VIII, XII and XIII have been identified ( ). Unlike the cornea, the scleral collagenous lamellae are irregularly arranged ( Fig. 1-17D ) and are interspersed with elastic fibres, each consisting of an elastin core surrounded by longitudinally arranged microfibrils composed of a number of glycoproteins including fibrillin. The opaque nature of the sclera, in contrast to the transparency of the cornea, can be partly ascribed to this irregular arrangement of the collagen fibres ( Fig. 1-17D ), but also to the variable fibre diameter (25–250 nm), variable and irregular fibrillar spacing, higher water content, and the reduced coating of GAGs on collagen fibres. Indeed, the sclera contains one-quarter of the proteoglycan and GAG content of the cornea. Dermatan sulphate and chondroitin sulphate proteoglycans are the most abundant in the sclera.
The yellowing of the eyeball in jaundice is the result of bilirubin deposition in the conjunctiva and not the sclera. Abnormal thinning of the sclera, such as occurs in some connective tissue disorders, e.g. Ehlers–Danlos syndrome, may also lead to a blue tinge. Localized thinning of the stromal collagenous layers may lead to staphyloma (bulging).
Collagen fibrils take up tensile force and are aligned with the direction of greatest tensile strength. The arrangements of scleral collagen can be studied using the ‘split-line’ technique, which has revealed that the collagen fibrils in the outer sclera are arranged in bundles that course in whorls, loops and arches, particularly around the muscle insertions and optic nerve ( Fig. 1-17C ). The collagen fibrils on the internal aspect of the sclera are arranged in a rhombic pattern ( Fig. 1-17D ).
The sclera extends anteriorly from the limbus to the lamina cribrosa posteriorly ( Fig. 1-17A and Fig. 1-42 ). The scleral collagen fibrils are arranged in circles or figure-of-eight patterns at the lamina cribrosa. Structures that transverse the sclera are shown in Figure 1-17A . Histologically the sclera has three layers: the lamina fusca, stroma and episclera ( Fig. 1-17B ).
The ‘blue’ sclera of infants is the result of the underlying choroidal pigment showing through the thin collagenous stroma. In elderly individuals, fat deposition in the sclera may produce a yellowish hue.
Limbus and aqueous outflow pathways ( Fig. 1-18 )
It is becoming increasingly appreciated that the limbus ( Fig. 1-18A–C ) is more than the border zone between the cornea and sclera; it has multiple functions including nourishment of the peripheral cornea, corneal wound healing, immunosurveillance of the ocular surface and hypersensitivity responses; it contains the pathways of aqueous humour outflow and is thus involved in the control of intraocular pressure. Surgical incisions to access the anterior chamber for cataract and glaucoma surgery are made at the limbus. The limbus is 1.5–2.0 mm in width and the change in the radius of curvature between the sclera and cornea produces a shallow external scleral sulcus and an internal scleral sulcus ; the latter is deepened by the scleral spur and houses the canal of Schlemm and trabecular meshwork. The longitudinal ciliary muscle fibres attach to the posterior aspect of the scleral spur , and its anterior surface gives rise to the corneoscleral trabeculae.

Several important transitions take place at the limbus ( Fig. 1-18A–C ).
- •
The regularly arranged corneal lamellae give way to the more random array of lamellae in the sclera. The corneal termination is V-shaped ( Fig. 1-18B,C ).
- •
The stratified squamous non-keratinized corneal epithelium with its parallel internal and external surfaces gives way to conjunctival epithelium, characterized by a folded basal surface and interdigitating subepithelial connective tissue (sometimes forming distinct papillae) ( Fig. 1-18B ).
- •
The conjunctival epithelium contains goblet cells and a rich network of MHC class II + CD11c + dendritic (Langerhans) cells (see section on conjunctiva, p. 83 ).
- •
Loops or arcades of conjunctival capillaries (derived from the anterior ciliary arteries) and lymphatic capillaries terminate at the limbus. The smaller vessels are not under neuronal control and are particularly susceptible to the effects of vasoactive amines (e.g. histamine, leukotrienes, prostaglandins) released by local immune cells (see below).
- •
Desçemet’s membrane and Bowman’s layer terminate in this region.
- •
The loose conjunctival subepithelial vascularized connective tissue (substantia propria), containing immunocompetent cell types such as mast cells, plasma cells and lymphocytes, tapers off at the limbus and is absent in the cornea ( Fig. 1-18B ).
Definitions of the limits and markings of the limbus vary among anatomists, pathologists and surgeons. The anatomical (histological) limbus is defined by a line that follows the V-shaped transition of corneal lamellae to scleral lamellae ( Fig. 1-18B ). Pathologists define the limbus as a block of tissue bordered anteriorly by a line passing through the termination of Schwalbe’s line and the junction of the conjunctival and corneal epithelium (corneolimbal junction), and posteriorly by a line from the scleral spur perpendicular to the tangent of the external surface. Surgeons usually cut close to the blue–grey transition zone seen on external examination, and incisions made here will pass anterior to the trabecular meshwork and Schlemm’s canal ( Fig. 1-18B,C ).
Aqueous outflow pathways
In the chamber or iridocorneal angle, partially nestled in the internal scleral sulcus, lies a complex wedge-shaped circumferential band of specialized, sponge-like, connective tissue, the trabecular meshwork , with the canal of Schlemm (sinus venosus sclerae) on its outer aspect ( Figs 1-18B,C and 1-19A ). The base of the trabecular meshwork is formed posteriorly by the scleral spur, the anterior face of the ciliary muscle and the iris root. The apex of the meshwork terminates anteriorly at Schwalbe’s line and the adjacent innermost corneal lamellae ( Figs 1-18 and 1-19A ). The trabecular meshwork can be further subdivided into three anatomical zones: the innermost uveal meshwork with cord-like trabeculae; the corneoscleral meshwork with flattened sheet-like trabeculae ( Fig. 1-19D ); and the outermost cribriform meshwork beneath the inner wall of Schlemm’s canal ( Fig. 1-19E ). The cribriform meshwork, unlike the rest of the trabecular meshwork, is not arranged in lamellae but consists of trabecular cells enmeshed in a loose extracellular matrix of collagen (types I, III and IV), elastic-like fibres and proteoglycans. This layer is thought to be the main site of resistance to aqueous outflow. The elastic cores of the trabeculae are continuous with the elastic fibres in the cribriform meshwork, which are in turn connected to the inner wall of Schlemm’s canal via ‘connecting fibres’. The anterior ciliary muscle fibres terminate in the elastic cores of the trabeculae. As the ciliary muscle contracts and moves inwards, the three-dimensional trabecular meshwork can be expanded, which results in an increase in the amount of ‘free’ spaces in the cribriform meshwork. This in turn allows greater aqueous outflow, thus increasing aqueous outflow facility.

Aqueous humour passes from the anterior chamber through the intertrabecular and intratrabecular spaces , which are lined by trabecular cells. These cells envelop the trabeculae ( Fig. 1-19D ) and maintain the state of hydration of the connective tissue core in a similar manner to corneal endothelium. In addition, trabecular cells are also phagocytic, trapping and removing debris from the aqueous humour as it percolates through the tortuous intertrabecular and intratrabecular spaces which narrow as Schlemm’s canal is approached ( Figs 1-18C and 1-19A ).
Schlemm’s canal ( sinus venosus sclerae ) is an endothelium-lined 36 mm long circumferential channel filled with aqueous humour. It measures 200–400 µm in the anteroposterior axis, is seldom more than 50–60 µm deep and is often septate. The canal is drained by 25–35 collector channels (20–90 µm in diameter) and between two and eight aqueous veins (of Ascher) (up to 100 µm in diameter). These either join deep, intrascleral and episcleral venous plexuses which drain into conjunctival veins or, in the case of aqueous veins, may drain directly into superficial conjunctival veins. The majority of aqueous humour (70–90%) leaves the anterior chamber through the trabecular meshwork and Schlemm’s canal ( ‘conventional’ outflow pathways ) . The inner wall of the canal is characterized, in well-preserved and properly fixed eyes, by transcellular channels or giant vacuoles ( Fig. 1-19B,C ). There is good evidence to suggest that these intracellular vacuoles, with openings on both the trabecular and luminal aspects, function to drain the great bulk of aqueous humour. The number and size of vacuoles and their openings or pores vary in a pressure-sensitive manner. Small quantities of aqueous may also pass between endothelial cells in the canal wall.
A proportion (10–30%) of aqueous humour drains via the ‘non-conventional’ aqueous outflow pathways. This route is not pressure sensitive and consists of the intercellular spaces between ciliary muscle fibres and the loose connective tissue of the suprachoroidal space. From here, aqueous traverses the sclera via the connective tissue sheaths of nerves and vessels that pierce its substance (see Fig. 1-17A ).
Glaucoma
Glaucoma is defined as a progressive optic nerve neuropathy. For most forms of glaucoma, elevated intraocular pressure and ageing remain important risk factors, although low tension or normal tension forms of glaucoma are common. However, in many forms of this condition pathological changes in the trabecular meshwork and Schlemm’s canal may be responsible for increased resistance to aqueous outflow and raised, or diurnal fluctuations in, intraocular pressure (IOP). In congenital glaucoma there is malformation of the complex three-dimensional arrangement of the trabeculae and excess extracellular matrix in the outer meshwork. Physical blockage of the inner surface of the chamber angle by the iris occurs in closed-angle glaucoma ; this may be a primary or a secondary process (see p. 512 ). Various forms of obstruction in the trabecular meshwork may give rise to open-angle glaucoma (see p. 512 ); the cause of the primary form of this condition is unknown, although there is evidence to indicate that excessive deposition of extracellular elements may occur in the cribriform meshwork. Secondary forms of open-angle glaucoma may be the result of debris such as lens proteins, melanin, macrophages and haemorrhagic products physically obstructing the intertrabecular and intratrabecular spaces, thus causing raised IOP (see p. 512 ).
Uveal tract or uvea
The uveal tract (L. uva = grape), the middle vascular pigmented layer of the eye, consists of the iris, ciliary body and choroid (see Fig. 1-10 ). These three components are continuous with one another and have an opening anteriorly, the pupil, and posteriorly the choroid is deficient at the optic nerve canal. The uveal tract is analogous to the vascular pia-arachnoid of the brain and optic nerve, with which it anastomoses at the optic nerve head. The choroid is described on p. 55 ; the iris and ciliary body are described below.
The iris
The iris ( Fig. 1-20A,B ) is a thin, heavily pigmented, contractile circular disk analogous to the diaphragm of a camera. It is suspended in the frontal or coronal plane anterior to the lens and ciliary body, and is surrounded by aqueous humour. The iris separates the anterior and posterior chambers, which are in continuity through an opening, the pupil , which lies slightly inferonasal to the centre of the iris. The iris is attached by its root at the angle (iridocorneal) of the anterior chamber where it merges with the ciliary body and trabecular meshwork. The free edge is known as the pupillary margin.

The iris is 12 mm in diameter with a circumference of 37 mm. It is cone-shaped with the pupil margin positioned more anteriorly than the root. The pupil margin rests on the lens, without whose support, for example in aphakic patients, it becomes tremulous (iridonesis). The size of the pupil regulates the amount of light entering the eye and is dependent on the state of contraction of the intrinsic pupillary muscles, the dilator and sphincter pupillae. The pupil may vary from 1 to 8 mm in diameter and there may be a slight degree of asymmetry between right and left eyes in normal individuals.
Structure ( Fig. 1-20A,B )
The pupil margin and iris root are thin, and hence more susceptible to tearing in contusion injuries (iridodialysis). The anterior surface is divided into two zones, the ciliary zone and pupil zone , by the thickened region known as the collarette. The anterior surface is characterized by radial streaks (straight when the pupil is contracted and wavy when dilated) and contraction furrows (more noticeable in dilated irides). The surface of the iris appears smooth in dark irides, in which the intrastromal melanocytes are heavily pigmented, and more irregular in blue irides, which have a less heavily pigmented stroma. The blue appearance of some irides is because of absorption of long wavelengths and reflectance of shorter wavelengths, especially by the collagenous stroma.
Microscopic anatomy
The iris consists of four layers: anterior border layer, stroma, dilator pupillae muscle and posterior pigment epithelium.
Anterior border layer ( Figs 1-20B and 1-21A ).
This layer is not covered by a layer of epithelial cells, as early anatomists believed, but is in fact made up of modified stroma consisting of a dense collection of fibroblasts, melanocytes and a few interspersed collagen fibres. This layer is deficient in areas; consequently the iris stroma is in free communication with the aqueous humour in the anterior chamber. Larger deficiencies in the anterior border layer are evident macroscopically as crypts. Aggregates of heavily pigmented melanocytes in this layer appear as naevi.

Stroma ( Fig. 1-21C–E ).
This consists of loose connective tissue containing fibroblasts, melanocytes and collagen fibres (types I and III). The loose nature of this tissue, and its free communication via openings in the anterior border layer, allows fluid to move in and out of the stroma quickly during dilation and contraction. The iris stroma in humans contains numerous mast cells and macrophages, many of which are perivascular (see Fig. 1-21C and Ch. 7 ). Many of the macrophages are heavily pigmented, and a subgroup may form large ovoid ‘clump cells’ (of Koganei), which tend to accumulate near the iris root and sphincter pupillae muscle ( Fig. 1-20B ). Lying free within the stroma close to the pupil margin is the sphincter pupillae muscle, a circumferential ring of smooth muscle fibres about 1 mm in width. The sphincter muscle consists of muscle bundles, each comprising six to eight smooth muscle cells, which are continuous via gap junctions and surrounded by a basal lamina. This muscle is innervated by parasympathetic nerve fibres derived from the oculomotor nerve (postganglionic fibres from the ciliary ganglion travel via the short ciliary nerves) although sympathetics also terminate in this muscle. The unusual embryological origin of this muscle from the neuroectoderm is described in Chapter 2 .
Dilator pupillae muscle.
This is a layer of myoepithelial cells derived from the anterior iris epithelium. The basal processes of this epithelium are 4 µm in thickness and extend up to 50–60 µm in a radial direction, while the apices of the myoepithelial cells are lightly pigmented and closely apposed to the apical aspect of the posterior pigment epithelium ( Figs 1-20B and 1-21A,E ). The dilator pupillae muscle is innervated by non-myelinated sympathetic fibres whose cell bodies are situated in the superior cervical sympathetic ganglion. Its parasympathetic innervation seems less significant. The dilator pupillae extends only as far centrally as the outer margin of the sphincter pupillae.
Posterior pigment epithelium ( Fig. 1-21E ).
This heavily pigmented layer consists of large cuboidal epithelial cells that appear black macroscopically on examination of the posterior surface of the iris. The posterior layer is derived from the inner neuroectodermal layer of the optic cup (see Ch. 2 ). The cells extend for a short distance on to the anterior iris surface at the pupillary margin; this forms the black ruff seen on the pupil margin during slit-lamp examination of the eye ( Fig. 1-20A ). The posterior pigmented epithelial layer forms a series of radially arranged furrows (most evident near the pupil margin) and circumferential contraction folds (most evident in the periphery).
Pupil movements
Mydriasis (dilation) occurs in conditions of low light intensity and in states of excitement or fear. It is a result of the action of the dilator pupillae muscle.
Miosis (contraction) occurs in more illuminated conditions, during convergence, and while sleeping. It is the result of the action of the sphincter pupillae muscle.
Blood supply of the iris
The iris has a rich blood supply and extensive anastomoses. At the root there is an incomplete major ‘circle’ of the iris that is derived from anterior rami of the anterior ciliary arteries. Branches from here pass centripetally and form an incomplete minor arterial ‘circle’ at the level of the collarette.
The arteries have an unusual coiled form to accommodate the variable states of contraction of the iris. Veins lie close to the arteries, with larger veins primarily in the anterior stroma and smaller veins in the deeper layers. Veins drain posteriorly/centrifugally into the ciliary body and eventually the vortex veins (see Fig. 1-39A,E ).
Iris capillaries are characterized by non-fenestrated endothelial cells that have a high density of endocytotic vesicles and tight junctions. This makes them less permeable to a variety of solutes than normal somatic vessels (hence they do not normally leak in fluorescein angiography). These vessels thus constitute an important component of the blood–ocular barrier. The basal lamina of the endothelial cells is thickened (0.5–3 µm) and further strengthened by perivascular collagenous/hyalinized layers ( Fig. 1-21A,B ). Periarteriolar smooth muscle cells are rare and elastic fibres are absent.
Nerve supply
The iris possesses a rich three-dimensional nerve plexus of myelinated and non-myelinated nerves. The sensory nerves are branches of the long and short ciliary nerves, themselves branches of the nasociliary nerve (ophthalmic division of the trigeminal). The autonomic innervation of the iris muscles is discussed above.
The ciliary body
The ciliary body ( Fig. 1-22A–D ) is an approximately 5–6 mm wide ring of tissue that extends from the scleral spur anteriorly to the ora serrata posteriorly. Temporally it measures 5.6–6.3 mm, and nasally 4.6–5.2 mm. It is divided into two zones, an anterior pars plicata (corona ciliaris) and a posterior pars plana ( Fig. 1-22A ). The ciliary body is approximately triangular in cross-section; its base faces the anterior chamber and the apex blends posteriorly with the vascular choroid. The pars plicata is 2 mm wide and consists of 70 radially arranged folds known as ciliary processes ( Fig. 1-22B ), each of which is 0.5–0.8 mm high and 0.5 mm wide. The tips are paler as a result of decreased pigmentation. Minor ciliary processes may be present in the valleys between the major processes. The pars plana is an approximately 4 mm wide zone stretching from the posterior limits of the ciliary processes to the ora serrata , the sharp serrated or dentate junction where non-pigmented ciliary epithelium undergoes a sharp transition to become the neural retina.

The ciliary body can be divided histologically into the ciliary epithelium, ciliary body stroma and ciliary muscle ( Fig. 1-22A ). Each is described below in the context of the three principal functions of the ciliary body: (1) accommodation; (2) aqueous humour production; and (3) production of lens zonules, vitreal glycosaminoglycans and vitreal collagen.
Accommodation
The anterior two-thirds of the ciliary body is occupied by the ciliary muscle which, in conjunction with the lens zonules (suspensory ligament) and the natural elastic nature of the lens fibres and capsule, functions to alter the refractive power of the lens. Histologically, the ciliary muscle in meridional sections consists of three groups of smooth muscle fibre bundles embedded in a vascular connective tissue stroma ( Figs 1-18 and 1-22A ). The stroma contains melanocytes, fibroblasts, and occasional immune cells such as mast cells, macrophages and lymphocytes.
The outer longitudinal muscle fibres are attached to the scleral spur and therefore indirectly to the corneoscleral trabeculae anteriorly; posteriorly they are anchored to the inner aspect of the sclera. The middle oblique or radial muscle fibres are continuous with inner corneoscleral trabeculae. The inner circular muscle fibres (one of the three so-called ‘Müller’s muscles’ associated with the eye and adnexa) appear as cross-sectional profiles in conventional meridional sections of the ciliary body ( Figs 1-18C and 1-22A ). A three-dimensional scheme has been proposed to explain the interrelationship between all three groups of muscle bundles; it appears that the fibres are all components of one interwoven fibre network (see Fig. 1-23 for summary).

The mode of action of the ciliary muscle is still controversial; however, it is generally agreed that during accommodation there is some degree of forward and inward shift of the ciliary body, which serves to slacken the tension on the zonules, thus increasing the refractive power of the lens (see pp. 35 and 217 ).
Action of the ciliary muscle on aqueous outflow.
There is some evidence that contraction of the longitudinal ciliary muscle fibres causes an inwards and posterior movement of the scleral spur as well as an effect via extensions of these tendons to the inner wall of Schlemm’s canal, hence distending the inter- and intratrabecular spaces of the trabecular meshwork and preventing collapse of Schlemm’s canal during periods of high pressure. These have been proposed as modes of action of miotic drugs such as pilocarpine, which are used to increase aqueous outflow facility in patients with glaucoma.
Production of aqueous humour
Aqueous humour is a clear colourless fluid actively secreted by the ciliary processes (for details of aqueous constituents see Ch. 5 ). The ciliary processes consist essentially of delicate finger-like protrusions of loose vascular connective tissue covered by a bilaminar cuboidal/columnar neuroepithelium, the outer pigmented and inner non-pigmented ciliary epithelium, both derived embryologically from the double neuroectoderm of the optic cup (see Ch. 2 ). The morphological basis of the blood–aqueous barrier is depicted in Figure 1-22B . Aqueous humour is actively secreted by the inner non-pigmented ciliary epithelium whose apices are connected by junctional complexes including tight junctions. Macromolecules, having filtered through the highly permeable fenestrated stromal capillaries, pass between pigment epithelium cells held together only by punctate adherentes (macula adherentes)-like junctions or permeable band-like junctions (zonula adherentes), and are prevented from passing into the posterior chamber. The non-pigmented ciliary epithelium has morphological features characteristic of secretory epithelium, namely numerous mitochondria, and histochemically can be shown to contain enzymes, such as carbonic anhydrase and ATPase, necessary for active fluid transport (see Ch. 4 , p. 217 ).
Production of zonules, vitreal collagen and vitreal hyaluronic acid
The non-pigmented ciliary epithelial cells of the pars plana are cuboidal, columnar or irregular, depending on age and location. It is likely that these cells play a role in secretion of zonular fibres, the extracellular vitreal components, i.e. collagen and hyaluronic acid, and the inner limiting membrane, especially during embryonic development.
Some inner non-pigmented cells ‘tilt’ anteriorly, suggesting traction by the zonular fibres. The lens zonules (of Zinn) or suspensory ligament of the lens merge with the fibrous basal lamina material of the non-pigmented ciliary epithelium ( Fig. 1-22C ). The precise mode of zonule synthesis is still unclear. Tall non-pigmented ciliary epithelial cells in the pars plana have ultrastructural and histochemical features that indicate active hyaluronic acid secretion.
Blood supply of the ciliary body
The blood supply of this region is derived from long posterior ciliary arteries and anterior ciliary arteries. The two long posterior ciliary arteries arise from the ophthalmic artery and after piercing the sclera near the optic nerve head they travel forward in the choroid in the medial and lateral horizontal plane and divide in the ciliary body before anastomosing with anterior ciliary branches, thus forming the major ‘circle’ of the iris (see p. 217 , and Fig. 1-36 ). From this circle arises muscular and recurrent choroidal branches and the numerous branches that form the vascular plexus in the ciliary processes. Venous return occurs predominantly posteriorly through the system of vortex veins and less so through the anterior ciliary veins.
Nerve supply of the ciliary body
The ciliary body has rich parasympathetic, sympathetic and sensory innervations.
Parasympathetic innervation.
Preganglionic neurone cell bodies are located in the Edinger–Westphal nucleus which lies posterior to the main oculomotor nucleus in the rostral midbrain at the level of the superior colliculus. Their axons travel in the oculomotor (III) nerve and synapse with postganglionic cell bodies located in the ciliary ganglion. The postganglionic fibres travel to the eye in the short ciliary nerves and terminate as an extensive plexus in the ciliary muscle. The action is mediated by acetylcholine on muscarinic receptors (see Ch. 6 , p. 355 ).
Sympathetic innervation.
Preganglionic neurone cell bodies are situated in the lateral grey horn of the first thoracic segment of the spinal cord. Preganglionic fibres relay in the superior cervical ganglion (adjacent to vertebrae C2 and C3, behind the internal carotid artery). Postganglionic fibres leave the ganglions as the internal carotid nerve and plexus. These fibres may reach the orbit as either direct branches of the internal carotid plexus or by joining the ophthalmic division of the trigeminal and its main branch in the orbit, the nasociliary nerve. Sympathetic fibres may either pass directly to the retrobulbar plexus behind the eye or through the ciliary ganglion uninterrupted. From the ciliary ganglions, fibres are distributed via the short ciliary nerves to the blood vessels of the eye, including the ciliary body. Some terminal filaments of the internal carotid plexus may also be distributed via the ophthalmic artery and its branches. The sympathetic action is mediated by the action of norepinephrine on two subclasses of receptors, α 1 and β 2 adrenoceptors, both of which are inhibitory (see Ch. 6 , p. 358 ).
Sensory innervation.
Sensory innervation is derived from the nasociliary nerve; however, the function of these fibres is unknown.
The lens and zonular apparatus
The lens ( Fig. 1-24 ) is a highly organized system of specialized cells (so-called lens ‘fibres’), which constitutes an important component of the optical system of the eye and fulfils the important function of altering the refractive index of light entering the eye to focus on the retina. While it has less refractive power (15 dioptres) than the cornea, the lens has the ability to change shape, under the influence of the ciliary muscle, and thus alter its refractive power. The range of dioptric power diminishes with age (8 at 40 years, 1–2 by 60 years). The transparency of the lens is due to the shape, arrangement, internal structure and biochemistry of the lens cells or lens fibres.

Position, size and shape
The lens, enclosed in its capsule, lies behind the iris and in front of the vitreous body. It is encircled by the ciliary processes and held in position by the zonular fibres laterally, the anterior vitreous face posteriorly (patellar fossa), and the iris anteriorly (see Fig. 1-10 ). It is normally transparent and avascular following regression of the pupillary membrane and tunica vasculosa lentis late in fetal development (see Ch. 2 ). It receives its nourishment from the aqueous and vitreous humours. It is a biconvex, ellipsoid structure with differing radius of curvature on the anterior and posterior surfaces. The anterior curvature is approximately 10 mm (range 8–14 mm) and the posterior curvature is approximately 6 mm (range 4.5–7.5 mm). The centre points of these surfaces, described as the anterior and posterior poles , are connected by an imaginary axis. The anterior pole lies 3 mm from the posterior corneal surface. The anterior and posterior surfaces are separated by the equator , which has a ridged (indented) appearance caused by the zonular fibres. In the adult eye the lens measures approximately 10 mm in diameter and has an axial length of 4 mm. The lens continues to grow (0.023 mm per year) and alters shape throughout life. It becomes rounder with age, especially after the age of 20 years.
Structure
The lens comprises three parts: (1) the capsule; (2) anterior or lens epithelium; and (3) the lens fibres ( Fig. 1-24A,D ).
Lens capsule.
The lens capsule is a thickened, smooth, basement membrane produced by the lens epithelium and lens fibres. It completely envelops the lens and has regions of variable thickness, being thickest pre- and post-equatorially (17–28 µm) and thinner at the posterior (2–3 µm) than at the anterior pole (9–14 µm). Ultrastructural examination reveals a fibrillar or lamellar appearance. The interfibrillar matrix consists of basement membrane glycoproteins (type IV collagen) and sulphated GAGs which are responsible for its prominent periodic acid–Schiff-positive staining properties in histological sections. It possesses elastic properties and, when not under tension of the zonules, the capsule together with the cortex causes the lens to assume a more rounded shape.
Lens epithelium.
This is a simple cuboidal epithelium ( Fig. 1-24A,D ) restricted to the anterior surface of the lens. The cells become more columnar at the equator. As they elongate, the apical portion comes to lie deeper to other, more anteriorly positioned, lens cells. These elongated lens cells are known as lens ‘fibres’ ( Fig. 1-24D ). The manner in which equatorial lens epithelial cells are transformed into lens fibres is depicted in Figure 1-24A . The cell nucleus and cell body sink deeper into the lens as further cells are laid down externally. Mitotic activity is maximal in the pre-equatorial and equatorial lens epithelium, known as the germinative zone ( Fig. 1-24A ).
Lens fibres.
While each lens fibre is only a 4 × 7 µm hexagonal prismatic band in cross-section ( Fig. 1-24C ), it may be up to 12 mm (12 000 µm) in length. The apical portion of the elongated lens cell (or lens ‘fibre’) passes anteriorly, the basal portion posteriorly. The cell nucleus migrates anteriorly as the cell is pushed deeper in the lens, hence creating the anteriorly oriented lens bow ( Fig. 1-24D ). The meridionally oriented lens fibres extend the full length of the lens, meeting at the anterior and posterior sutures ( Fig. 1-24A ). Deeper (hence older) lens fibres are anucleate. Continual growth of the lens, by addition of superficial strips of new cells, produces a series of concentrically arranged laminae, similar to the layers of an onion (best seen by dissecting a fixed or frozen lens). In life, the outer cortex of the lens has a softer consistency than the hard central nucleus.
Lens fibres are tightly packed with little intercellular space. Neighbouring cells are linked by ball-and-socket cytoplasmic interdigitations ( Fig. 1-24B,C ) and numerous gap junctions. The junctions may aid maintenance of centrally positioned cells (via intercellular and molecular coupling or metabolic cooperation) some distance from the source of nutrition (aqueous humour). Superficially located lens fibres are rich in ribosomes, polysomes and rough endoplasmic reticulum, and actively synthesize unique lens proteins, lens crystallins (see Ch. 4 ); however, the cytoplasm of mature lens fibres appears homogeneous. Lens fibres are rich in cytoskeletal elements oriented parallel to the long axis of the cell.
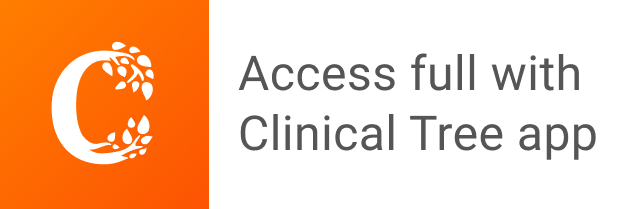