Fig. 2.1
Light (a) and transmission electron (b–h) micrographs of anatomical corneal layers. (a) Semithin cross section of the cornea showing three cellular layers, i.e., epithelium, stroma with keratocytes, and endothelium. (b) Corneal epithelium showing three layers, the superficial or squamous cell layer, the suprabasal wing cell layer, and the basal columnar cell layer resting on Bowman’s layer. (c) Anchoring complexes formed by hemidesmosomes (arrows) and anchoring fibrils (arrowheads) mediating attachment of basal epithelial cells to basement membrane (BM) and Bowman’s layer. (d) Bowman’s layer representing the most anterior portion of the corneal stroma. (e) Interface (dotted line) between Bowman’s layer and corneal stroma showing differing arrangement of collagen fibrils. (f) Descemet’s membrane (DM), the basement membrane of the corneal endothelium, being composed of interfacial matrix (IFM), anterior banded layer (ABL), and posterior nonbanded layer (PNBL). (g) “Bowman’s-like layer” (BL), a meshwork of randomly arranged collagen fibrils at the interface between Descemet’s membrane and stroma. (h) Connecting collagen fibrils (arrows) projecting from “Bowman’s-like layer” into the interfacial matrix zone (IFM) of Descemet’s membrane (magnification bars = 100 μm in a; 15 μm in b and d; 5 μm in f; and 0.5 μm in c, e, h) (e, g Reproduced from Schlötzer-Schrehardt et al. [66], and h Reproduced from Schlötzer-Schrehardt et al. [64], with permission from Elsevier)
Confocal microscopy with the Heidelberg retina tomograph (HRT) II and Rostock Cornea Module can be used for in vivo imaging of all anatomical layers and corneal cell types including nerve plexi and immune cells (Fig. 2.2).
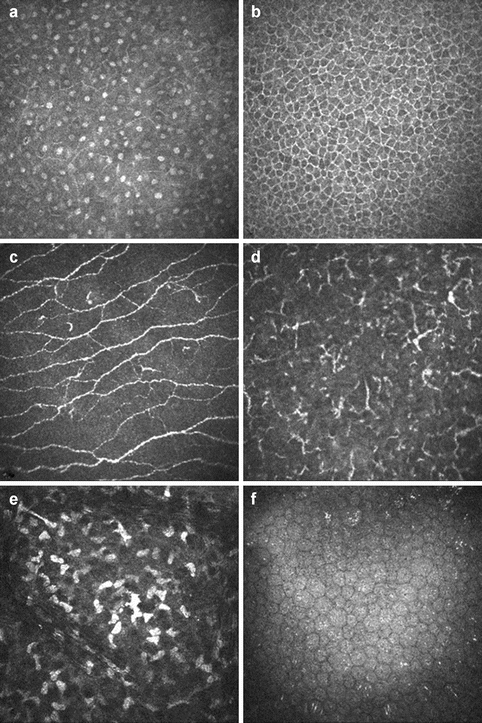
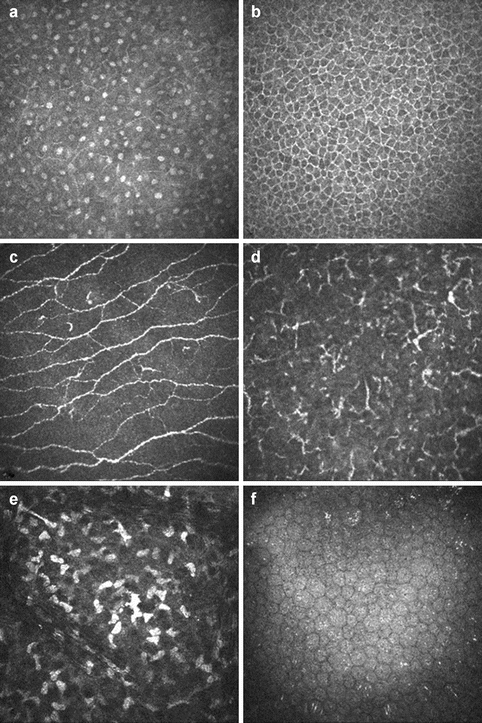
Fig. 2.2
In vivo confocal microscopy of corneal layers using the Heidelberg retina tomograph (HRT) II and Rostock Cornea Module in a 52-year-old patient at the levels of suprabasal epithelium (a), basal epithelium (b), subbasal nerve plexus (c), intraepithelial dendritic cells (d), stromal keratocytes (e), and corneal endothelium (f) (By courtesy of Christina Jacobi, Erlangen)
Corneal Epithelium
The epithelial surface of the cornea represents the physical barrier to the outer environment and an integral part of the smooth tear film–cornea interface that is critical for the refractive power of the eye. It is responsible for protecting the eye against loss of fluid and invasion of foreign bodies and pathogens and for absorbing oxygen and nutrients from the tear film.
The corneal surface is covered by a stratified, nonkeratinizing, squamous epithelium, about 50 μm in thickness, comprising 5–7 cell layers collectively. It can be structured into three layers, the superficial or squamous cell layer, the suprabasal wing cell layer, and the basal columnar cell layer (Fig. 2.1b) [18, 60]. Desmosomes promote strong adhesion between cells of all epithelial layers. The superficial layer is formed by 2–3 layers of flat polygonal cells, which form intercellular tight junctions to provide an effective barrier and numerous surface microvilli, which increase the cellular surface area and enhance oxygen and nutrient uptake from the tear film. The microvillar glycocalyx coat interacts with and helps to stabilize the pre-corneal tear film, which is composed of three layers: a superficial lipid layer to provide protection from evaporation, an aqueous layer providing nutrients and oxygen supply to the corneal epithelium, and a basal mucin layer, which interacts closely with the epithelial cell glycocalyx to allow lubrication of the ocular surface and spreading of the tear film with each eyelid blink [26, 70]. The tear film also supplies immunological and growth factors that are critical for epithelial health, proliferation, and repair, and defects in tear film, e.g., in neurotrophic keratopathy after corneal surgery, can cause epithelial wound healing problems and surface inflammation. The wing cell layer is formed by 2–3 layers of wing-shaped cells which have laterally interdigitated cell membranes with numerous desmosomes (Fig. 2.2a). The basal layer consists of a single layer of columnar cells (Fig. 2.2b), which are attached to the underlying basement membrane by hemidesmosomes (Fig. 2.1c). The epithelial basement membrane has a critical role in corneal wound healing, because defects in this delicate layer allow penetration of growth factors from the epithelium into the stroma [71]. Corneal epithelial adhesion to Bowman’s layer is maintained by an anchoring complex including anchoring fibrils (type VII collagen) and anchoring plaques (type VI collagen) (Fig. 2.1c) [25]. Abnormalities in these anchoring complexes may result clinically in recurrent corneal erosions or nonhealing epithelial defects.
Besides epithelial cells, there are numerous nerve endings in between the cells (Fig. 2.2c), which exert important trophic influences on the corneal epithelium and which have been estimated to amount to a density of 7000 nociceptors per mm2, which is 400 times more than in the skin [52]. Mechanical stress to these nerves, such as in bullous keratopathy, can therefore cause tremendous pain. Furthermore, resident MHC class II-expressing cells, i.e., CD11c+-dendritic cells and CD207+-Langerhans cells, were identified in the human basal epithelium and anterior stroma (Fig. 2.2d), which are capable of rapidly mobilizing to the site of epithelial trauma and viral infection within the cornea [37]. The corneal epithelium itself exerts strong anti-inflammatory and antiangiogenic properties, and transplantation of donor corneas without the epithelium, e.g., after abrasion, leads to increased postoperative inflammation and neovascularization [17].
Corneal epithelial cells routinely undergo apoptosis and desquamation from the surface. This process results in complete turnover of the corneal epithelial layer every 5–7 days as deeper cells replace the desquamating superficial cells in an orderly, apically directed fashion. Two populations of cells, the basal epithelial cells and limbal stem cells, help renew the epithelial surface [7]. The epithelial stem cells and their progenitors are located at the bottom of the palisades of Vogt at the corneoscleral limbus [15]. Depletion of this stem cell reservoir, e.g., after chemical burns, can cause severe ocular surface disease and significant visual deterioration, a condition known as limbal stem cell deficiency [1]. In these cases, epithelium of conjunctival phenotype may replace the corneal surface. Transplantation of limbal autografts or allografts [35] and ex vivo expanded limbal epithelial stem cells are established therapeutic strategies to regenerate the damaged corneal surface [67].
The corneal epithelium responds to injury in three phases, i.e., migration, proliferation, and differentiation with reattachment to the basement membrane [80]. Following injury, cells adjacent to an epithelial defect migrate to cover the wound within few hours. Following wound closure, basal epithelial and limbal stem cells proliferate and differentiate to repopulate the epithelium. In the final phase, hemidesmosomes replace focal contacts in order to anchor the basal epithelial cells tightly to the basement membrane and stroma. If the basement membrane remained intact, a tight adhesion is established in only a few days. If the basement membrane was damaged, its repair can take up to 6 weeks. During this time, the epithelial attachment to the newly deposited basement membrane tends to be unstable and weak, and the regenerated epithelium is very susceptible to damage. Following PKP, re-epithelialization is usually observed within 1 week, although morphological abnormalities, detected by specular microscopy, may persist up to 6 months postoperatively [74]. Corneal grafts showed some recovery of the subbasal nerve plexus, at least in the graft periphery, but not complete recovery of function [68].
Bowman’s Layer
Bowman’s layer represents the most anterior, acellular portion of the corneal stroma (Fig. 2.1d). It is approximately 8–12 μm thick and structurally composed of randomly oriented collagen fibrils, 20–25 nm in diameter, consisting of collagen types I, III, V, and VI (Fig. 2.1e) [77]. Its thickness has been reported to decline with age by 0.06 μm per year, thus losing one-third of its thickness between 20 and 80 years of age [23]. Unmyelinated nerve axons penetrate Bowman’s layer to terminate within the epithelium. The functional role of Bowman’s layer is not completely known, but it is believed to serve as a barrier that protects corneal stroma and nerves from traumatic injury. In addition, it has been suggested to ensure epithelial anchorage to the corneal stroma and helps to maintain the shape and tensile strength of the cornea. Bowman’s layer also functions as an important UV shield protecting the inner eye and a nearly insurmountable barrier against the invasion of epithelial tumors into the corneal stroma [60].
When disrupted, Bowman’s layer does not regenerate but forms a scar. Therefore, diseases or surgical procedures leading to defects in Bowman’s layer increase the risk for corneal ruptures and ectasias. On the other hand, sutures have to extend through Bowman’s layer to ensure tight and effective suturing [17].
Corneal Stroma
The stroma is the thickest layer of the cornea measuring approximately 500 μm in width and represents a dense avascular connective tissue of remarkable and unique regularity. It is composed of regularly arranged bundles of collagen fibrils embedded in a glycosaminoglycan-rich extracellular matrix, which are interspersed with flattened fibroblast-like cells termed keratocytes [18]. Collagen organization in the stroma is crucial to corneal functions such as light transmission and maintenance of corneal curvature, tensile strength, and rigidity [27]. The individual collagen fibrils, being mainly composed of collagen types I and V, are extremely uniform in diameter measuring about 25–30 nm [38, 44] and are organized into approximately 250–300 2 μm thick sheets or lamellae. Regular spacing of fibrils within these lamellae is maintained by interactions of collagens with proteoglycans forming bridges between the fibrils [53]. The major proteoglycans of the stroma are keratan sulfate proteoglycans, such as keratocan and lumican, and chondroitin/dermatan sulfate proteoglycans, such as decorin [27, 48], which also regulate stromal hydration by means of their ability to bind water molecules. The collagenous lamellae form a highly organized ply, with adjacent lamellae being oriented at right angles, although there are organizational differences in the collagen bundles between anterior and posterior stroma [45]. In the anterior third of the stroma, lamellae are oriented more obliquely, mediating a tighter cohesive strength and rigidity, which appears particularly important in maintaining corneal curvature [51], whereas in the posterior two-thirds, lamellae run in parallel to the corneal surface. These differences in stromal collagen organization may also explain why the anterior stroma resists changes to stromal hydration much better [46] and why surgical dissection in a particular plane is easier in the posterior depths of the stroma, e.g., in DALK. Moreover, the peripheral stroma is thicker than the central stroma, and the collagen fibrils may change direction to form a circumferentially oriented network, which is thought to be pivotal in maintaining corneal stability and curvature, as they approach the limbus [45]. Any disturbance of this fine-tuned arrangement, either by deposition of abnormal extracellular matrix, e.g., deposition of mucopolysaccharides in macular corneal dystrophy, or the irregular arrangement of collagen fibrils in stromal scars, can cause corneal opacity.
The collagen lamellae are interspersed with flattened stellate keratocytes, which are interconnected by gap junctions and arranged in a circular, corkscrew pattern forming a coherent network (Fig. 2.2e) [50, 59]. The density of keratocytes in the anterior stroma is 20,000–24,000 cells/mm2 and the density decreases posteriorly. Keratocytes are metabolically active cells involved in synthesis and turnover of extracellular matrix components, i.e., collagen molecules and glycosaminoglycans. They contain water-soluble proteins, corneal “crystallins,” which appear to be responsible for reducing backscatter of light from the keratocytes and for maintaining corneal transparency [32]. In addition, sensory nerve fibers are present in the anterior stroma, which are cut during PKP leading to a mild neurotrophic keratopathy [68], and MHC class II antigen-presenting cells, which seem to migrate out of the cornea during organ preservation, thereby explaining the reduced rates of immune rejections of longer organ-cultured grafts [17].
Following injury to the stroma, e.g., in PKP, keratocytes adjacent to the wound undergo apoptosis [71, 78, 80]. About 24 h after wounding, the remaining keratocytes begin to proliferate and transform into activated fibroblasts, which migrate into the wound region and produce extracellular matrix components, a process that may last up to 1 week. Inflammatory cells, including monocytes, granulocytes, and lymphocytes, infiltrate the stroma from the limbal blood vessels. Fibroblasts transform into myofibroblasts, which contract the wound and secrete extracellular matrix, a process which may last up to 1 month. Deposition of large amounts of disorganized extracellular matrix may lead to loss of corneal transparency causing stromal haze. Matrix remodeling by repopulating keratocytes thereby restoring transparency is the last phase of stromal wound healing and can last for years [71]. In penetrating or lamellar keratoplasty, a rather complete wound healing response is usually noted at donor-recipient interfaces. However, abnormal collagen fiber size and arrangement, indicating incomplete stromal wound remodeling and persistence of fibrotic scar tissue, have been observed within the graft margin after PKP [11]. Similarly, the presence of fibrocellular tissue, probably derived from myofibroblasts, has been found in the graft–host interface in about 20 % of corneas after DSAEK failure [79]. Therefore, stroma-to-stroma interface haze may occur in DALK or DSAEK and can degrade visual acuity, even if the microkeratome or femtosecond laser is used to achieve a smooth resection [4].
Recently, the existence of a novel, previously unrecognized layer of the pre-Descemet’s corneal stroma, which can be separated by air injection into the stroma during DALK using big-bubble technique, has been reported [19]. This distinct layer was reported to measure about 10 μm in width and was characterized to lack any keratocytes and to show a pronounced immunostaining for collagen types III, IV, and VI [20]. However, the description of this hypothesized new anatomic layer was critically commented on in the literature and eventually refuted by a detailed ultrastructural reinvestigation of the human corneal stroma [66]. The findings of this three-center study provided evidence that there is no distinctive acellular pre-Descemet’s stromal zone justifying the term “layer” apart from a thin (0.5–1.0 μm) intermediary “Bowman’s-like zone” of randomly arranged collagen fibers at the Descemet’s membrane–stromal interface (Fig. 2.1g). The collagen fibers of this intermediary layer partly extend into Descemet’s membrane serving a connecting function (Fig. 2.1h). Stromal keratocytes were found to approach Descemet’s membrane up to 1.5 μm (mean 4.97 ± 2.19 μm) in the central regions and up to 4.5 μm (mean 9.77 ± 2.90 μm) in the peripheral regions of the cornea. The intrastromal cleavage plane after pneumodissection, which seemed to occur at multiple stromal levels along rows of keratocytes offering the least resistance to mechanical forces, was obviously determined by the variable distances of keratocytes to Descemet’s membrane. Consistently, the residual stromal sheet separated by air injection into the stroma varied in thickness from 4.5 to 27.5 μm, being usually thinnest in the central and thickest in the peripheral portions of the bubble (Fig. 2.3d). This phenomenon has been well documented as “residual stroma” in previous studies, providing evidence that the big-bubble technique in DALK is not consistently a Descemet-baring technique [31, 36, 43].
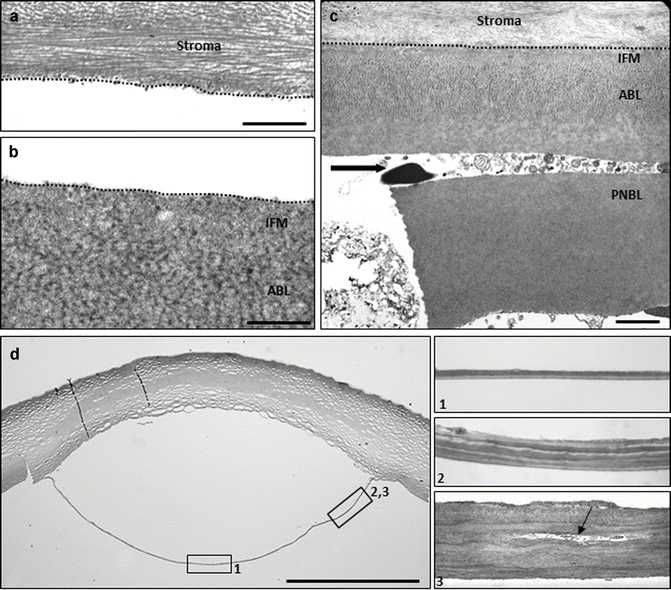
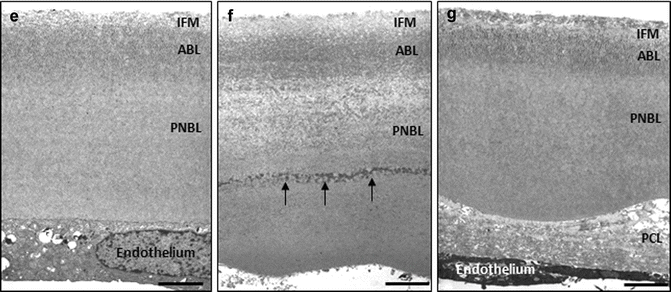
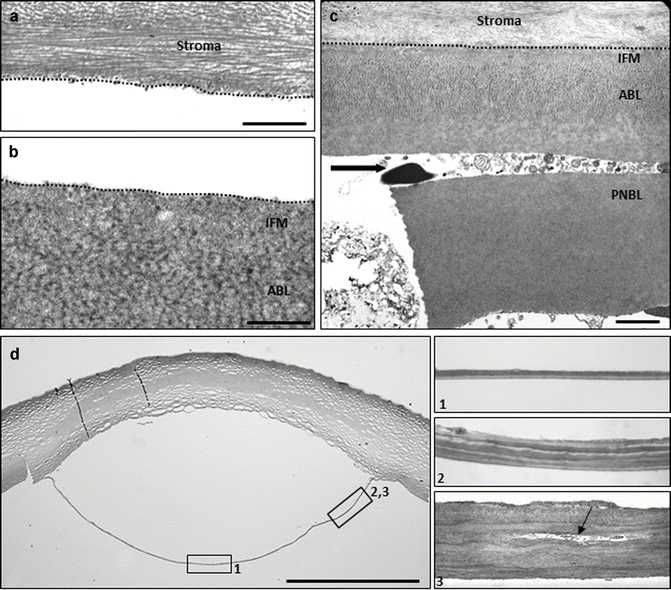
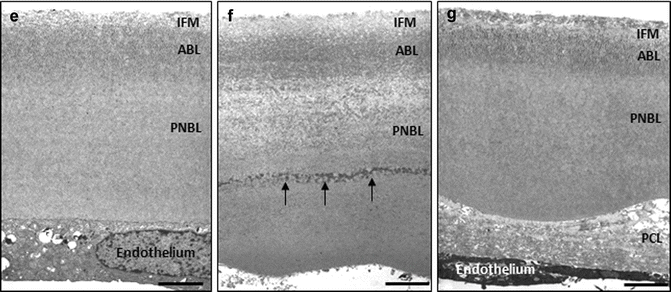
Fig. 2.3
Light (D) and transmission electron (a–c, e–g) micrographs showing cleavage planes in lamellar keratoplasty and usability of Descemet’s membrane ultrastructure as indicator of endothelial function. (a, b) Physiological cleavage plane between the posterior stromal collagen lamellae (a) and interfacial matrix zone (IFM) of Descemet’s membrane in DMEK. (c) Lamellar splitting of Descemet’s membrane between anterior banded layer (ABL) and posterior nonbanded layer (PNBL) (arrow) of a donor cornea with unsuccessful stripping due to strong adhesion of Descemet’s membrane to the corneal stroma (dotted line). (d) Semithin section of a donor cornea showing big-bubble formation after air injection into the corneal stroma; the boxed areas (1, 2, 3) are shown in higher magnification on the left illustrating the stromal sheet forming the bubble wall of variable thickness with remnants of keratocytes (arrow). (e–g) Ultrastructural analysis of Descemet’s membrane showing normal structure (e), abnormal collagen inclusions (arrows) within posterior nonbanded layer (PNBL) (f), and a posterior collagenous layer (PCL) deposited onto a normal Descemet’s membrane (g) (IFM interfacial matrix, ABL anterior banded layer; magnification bars = 2 mm in d; 2.5 μm in c, e, f, g; and 1 μm in a and b) (a, c, e reproduced from Schlötzer-Schrehardt et al. [65], and c reproduced from Schlötzer-Schrehardt et al. [66], with permission from Elsevier)
Descemet’s Membrane
Descemet’s membrane represents the thickened (10–12 μm), specialized basement membrane of the corneal endothelium consisting of collagen types IV, VIII, and XVIII and non-collagenous components including fibronectin, laminin, nidogen, and perlecan as well as dermatan, keratan, heparan, and chondroitin sulfate proteoglycans [64]. Apart from providing structural integrity of the cornea, Descemet’s membrane has been suggested to play a role in several important physiological processes including corneal hydration, endothelial cell differentiation and proliferation, and maintenance of the corneal curvature. It is composed of an anterior banded (fetal) layer, approx. 3 μm in thickness, and a posterior nonbanded (postnatal) layer that gradually thickens with age reaching up to 10 μm in elderly individuals (Fig. 2.1f) [33, 54]. In the periphery, Descemet’s membrane forms wart-like excrescences (Hassall-Henle warts) and merges into the trabecular meshwork beams. The thickened fusion site, known as Schwalbe’s line, is a gonioscopic landmark that defines the end of Descemet’s membrane and the beginning of the trabecular meshwork.
Descemet’s membrane is attached to the corneal stroma by a narrow (about 1 μm thick) transitional zone of amorphous extracellular matrix termed the “interfacial matrix,” which contains increased amounts of adhesive glycoproteins such as fibronectin (Fig. 2.1f, g) [64]. Connecting collagen fibers projecting from the “Bowman’s-like” stromal layer into this interfacial matrix zone further promote anchorage (Fig. 2.1h). Extracellular matrix complexes formed by keratoepithelin (transforming growth factor β-induced) and collagen type VI are also involved in maintaining adherence at Descemet’s membrane–stroma interface. Adhesive forces appear to be slightly stronger in the central than in the peripheral parts of the cornea. Nevertheless, Descemet’s membrane can be separated relatively easily from the adjacent stroma, which is utilized during DMEK surgery by a transient splitting of the physiological interface between the interfacial matrix of Descemet’s membrane and posterior stroma in both the donor’s and recipient’s corneas (Fig. 2.3a, b). The high optical and structural quality of this interface remains after reattachment of the donor’s Descemet’s membrane to the recipient’s corneal stroma, allowing for superior functional results after DMEK when compared to other lamellar transplantation techniques producing a stroma–stroma interface [72]. Although Descemet’s grafts can be manually prepared from donor corneas with a high level of reproducibility (98 %) using an appropriate technique [40], a small percentage of donor corneas (2 %) reveals individual tissue properties, which may complicate and even prevent proper Descemet’s stripping due to exceptionally strong adhesiveness of Descemet’s membrane to the posterior stroma [65]. The morphological cause underlying the resistance of Descemet’s membrane to proper stripping appears to be ultrastructural or biochemical abnormalities along Descemet’s membrane–stroma interface, and any attempts to strip Descemet’s membrane result in its lamellar splitting, mostly between anterior banded and posterior nonbanded layers (Fig. 2.3c). Lamellar splitting can also occur during stripping of recipient Descemet’s membrane, particularly in patients with Fuchs’ dystrophy leaving residual fetal Descemet’s membrane retained on the recipient DSAEK or DMEK interface [13, 49]. This phenomenon may be one frequent cause for failure of graft adherence to the recipient posterior corneal surface [76].
DMEK is dependent on the biomechanical elastic properties of Descemet’s membrane, which scrolls up with the endothelium on the outside upon removal from the stroma. Age, which is known to correlate with thickness of Descemet’s membrane [54], has a significant impact on the degree of scrolling. Thinner grafts from younger donors (<50 years) have a tendency for pronounced curling after stripping making subsequent unfolding in the recipient’s anterior chamber more difficult. Thus, corneas from donors older than 55 years of age are preferably used for DMEK graft preparation [41]. The exact reasons why grafts adhere to the recipient bed are not known. Physical, biochemical, and physiological mechanisms such as endothelial pump function have been proposed. It has been shown that the use of organ-cultured grafts exhibiting modified biochemical properties and a larger removal of Descemet’s membrane of the host promote graft adhesion [42, 73].
Although intraoperative manipulation may be a frequent cause of primary graft failure, the majority of failed DMEK grafts revealed ultrastructural signs of preoperative endothelial dysfunction, i.e., inclusions of abnormal collagenous material within Descemet’s membrane proper [14]. Due to its continued appositional growth with age [54], Descemet’s membrane provides a lifelong record of pathological events and endothelial function [33], and any deposition of abnormal extracellular material is indicative of previous stress or damage to the endothelial cells (Fig. 2.3e, f). Thus, a preexisting subclinical corneal endothelial dysfunction, as indicated by abnormal inclusions within Descemet’s membrane, may have contributed to primary DMEK failure [14]. In contrast, a posterior fibrous layer, mainly consisting of collagen types I and IV and fibronectin, may be produced and deposited on the posterior surface of an otherwise normal Descemet’s membrane by attenuated endothelial cells that underwent transdifferentiation into (myo)fibroblast-like cells (Fig. 2.3g) [75]. The formation of an abnormal posterior collagenous layer is the result of a final common pathway following endothelial dysfunction and damage, including intra- or postoperative trauma, and has been also reported to contribute to failed lamellar and penetrating grafts [28, 39].
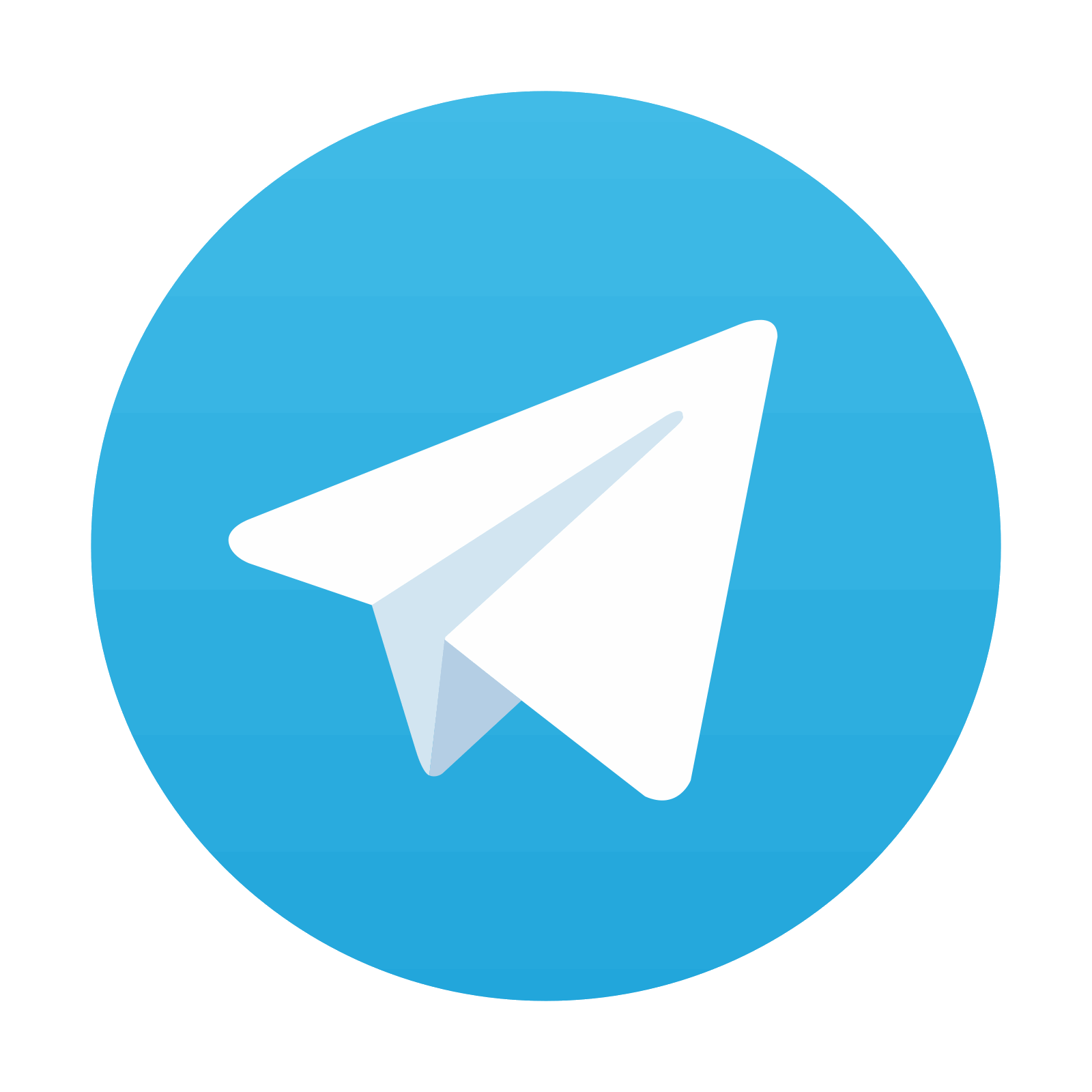
Stay updated, free articles. Join our Telegram channel
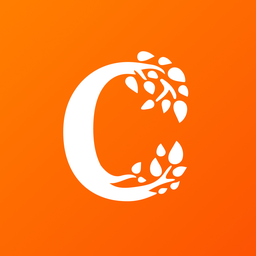
Full access? Get Clinical Tree
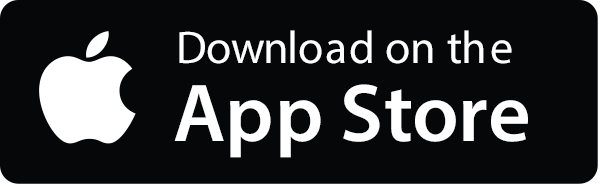
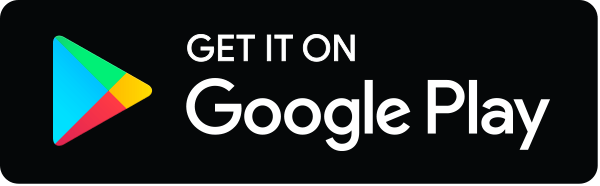