An Introduction to Color Vision
William E. Benson
ELECTROMAGNETIC ENERGY
The basic building block of matter, the atom, consists of a nucleus (composed of protons and neutrons) and electrons, which revolve around the nucleus (Fig. 1). The electrons do not revolve at random distances from the nucleus but in orbits of approximately fixed diameters. An electron can move from its orbit to a higher one if it receives energy from an external source (e.g., a flying electron, heat). However, the electron remains in the higher orbit for only one hundred-millionth of a second. As it falls back to a lower orbit, it releases its excess energy by emitting a small packet of energy called a quantum or a photon (Fig. 2). The farther the electron falls to reach its original lower orbit, the greater the energy of the photon.
In a vacuum, all photons move at the speed of light. As they travel, they vibrate, causing measurable electric and magnetic effects alongside their line of travel. Photons can be characterized by their energy; by their frequency of vibration (in v/second); and by their wavelength (λ), the straight-line distance a photon moves during one complete vibration. The higher the energy in a photon, the faster it vibrates. Frequency and wavelength are related by the formula f × λ = c, where f is frequency of vibration, λ is wavelength, and c is speed of light (approximately 3 × 108 m/second). Rewriting this as f = c/λ, we see that f and λ are inversely proportional (i.e., as frequency increases, wavelength decreases). For example, gamma rays have a very high frequency and a very short wavelength; radio waves have a very low frequency and a long wavelength.
Photons are usually classified according to their wavelength. The full range of photon energy is called the electromagnetic spectrum (Fig. 3). Light, x-rays, gamma rays, and radio waves are all forms of electromagnetic energy. The reason we can “see” light but not other forms of electromagnetic energy is that the rods and cones of the retina contain pigments that preferentially absorb photons with wavelengths between 400 and 700 nm (a nanometer is a billionth of a meter) and convert their energy into an electrical reaction (neuronal impulse) that is carried to the brain. Wavelengths longer than 700 nm and shorter than 400 nm tend to pass through the sensory retina without being absorbed (Fig. 4). Actually, the retina is capable of seeing photons of shorter wavelengths (ultraviolet light), but the cornea and lens filter them out. A person who is aphakic may be able to see ultraviolet light.
Light quanta can be characterized not only by wavelength but also by the sensation they cause when they strike the retina. Quanta of shorter wavelengths are perceived as magenta, blue, and green; those of a longer wavelength are perceived as yellow, orange, and red (Fig. 5).
Electromagnetic quanta travel at the speed of light only in a vacuum. If they enter any other medium, such as glass, their wavelength and speed decrease (Fig. 6). The frequency of vibration remains the same. The shorter the wavelength, the more the speed is decreased. For example, imagine two photons, one of wavelength 700 nm and the other of wavelength 400 nm, traveling through a vacuum. As long as they remain in a vacuum they keep pace with one another. If they now strike a piece of glass perpendicularly, the photon of 400 nm is slowed down more than the photon of 700 nm and falls behind. When they emerge from the glass they resume the speed of light and their original wavelength, but the 400-nm photon remains an instant behind. If they enter the medium obliquely, their paths are bent. The shorter the wavelength, the greater its path is bent. These phenomena explain why a prism breaks up white light into the colors of the spectrum (Fig. 7).
![]() Fig. 6. Photons traveling in a medium other than a vacuum are slowed in inverse proportion to their wavelength. |
RETINAL ORGANIZATION
In nature, vitamin A is in its all-trans, alcohol variant (Fig. 8). Unbleached rhodopsin, the visual pigment of rods, is made up of retinal, the 11-cis-aldehyde variant, and a protein called an opsin (Figs. 9 and 10). When light strikes a molecule of rhodopsin, 11-cis retinal is converted back to the all-trans form and is released from the opsin (Fig. 11), initiating an electrical impulse in the photoreceptor that travels toward the brain. The eye then resynthesizes the rhodopsin. Rods function primarily when the eye is dark adapted (i.e., for night vision) and cannot distinguish one color from another. Cones, on the other hand, function when the retina is light adapted (i.e., for day vision). Our ability to distinguish different colors depends on the fact that there are three different kinds of cone, each of which has a different visual pigment. All cone pigments contain 11-cis retinal. They differ in that each has a different opsin. The function of the different opsins is to rearrange the electrons of retinal, thereby changing its ability to capture photons of different wavelength.
Red-catching cones (R cones) contain a pigment referred to by Rushton as erythrolabe, which preferentially absorbs quanta of longer wavelengths. It is best stimulated by light of a wavelength of 570 nm, but it also absorbs adjoining wavelengths (Fig. 12). Green-catching cones (G cones) contain chlorolabe, which is best stimulated by intermediate wavelengths. Its maximal sensitivity is to a wavelength of 540 nm. Blue-catching cones (B cones) contain cyanolabe, which absorbs the short wavelengths best. Its maximal sensitivity is at 440 nm.
The retina is organized so that it sends to the brain an encoded description of the light that strikes it. The initial step is the absorption of photons by the pigments of the photoreceptors (rods and cones) (Fig. 13). The electrical signals are transmitted at connections called synapses to bipolar cells and, subsequently, to ganglion cells. Horizontal cells receive information from many rods and cones and modify these messages before they are sent toward ganglion cells (Fig. 14). For example, if a cone is strongly stimulated, it sends inhibitory messages by way of a horizontal cell to neighboring cones. Bipolar cells send inhibitory messages by way of amacrine cells. The axons of ganglion cells form the optic nerve, which carries information to the brain. The “hue center” in the brain adds up the information from the different color channels and determines which color we see. In general, the hue we see depends on the relative number of photons of different wavelength that strike the cones.
COLOR PERCEPTION
To accurately describe any color, one must specify three attributes: hue, saturation, and brightness. Hue is the attribute of color perception denoted by blue, red, purple, and so forth. Hue depends largely on what the eye and brain perceive to be the predominant wavelength present in the incoming light. In simplest terms, this means that if light of several wavelengths strikes the eye and if more light of 540 nm is present than is light of other wavelengths, we will see green (Fig. 15). However, what the brain perceives depends on many other factors, which need further elaboration.
The color wheel is made up of all hues arranged in a continuous circle such that each hue lies between those hues it most closely resembles. Complementary hues lie opposite each other (Fig. 16). Using the color wheel, we can predict the color that will result when two different lights are mixed. There are two basic rules. First, when equal quantities of complements or equal quantities of all wavelengths are mixed, the result is white. Second, when noncomplements are mixed, the resultant color lies between the two original colors (Demonstration 1).* The exact color seen depends on the quantity of each color used. For example, equal quantities of red and green result in yellow, whereas a large quantity of red and a relatively small quantity of green result in orange. Unlike the ear, which can distinguish several musical instruments playing at once, our eye and brain cannot determine which wavelengths of light are present in the color we see. For example, if we present the eye with a light composed purely of wavelength 589 nm, the eye sees it as yellow. However, if light of wavelength 546 nm (green) and light of wavelength 689 nm (red) are mixed in the proper proportions, the eye sees what looks to be the same yellow. It does not perceive that this yellow is composed of red and green light and that no light of 589 nm is present. Similarly, when two complements are mixed, we see white and cannot distinguish this white from the white seen when equal quantities of all wavelengths are present. Further, if we add a small amount of all wavelengths from 400 to 700 nm either to a pure yellow composed only of wavelength 589 nm or to the mixture of red and green, the eye still sees yellow. It cannot determine that additional wavelengths are present in the incoming light.
When we see light composed only of wavelength 490 nm, we call it blue green, as if blue and green light are both present, even though only one wavelength is present. We cannot distinguish this color from an appropriate mixture of blue and green. The same analysis applies to all other nonprimary spectral colors (orange, yellow, yellow green, and blue green). All are perceived when the proper wavelength or the proper mix of red, green, and blue is present (Demonstration 1).
When speaking of colored lights, the primary hues (also called the additive primaries) are red, green, and blue. These three hues, when added together in the proper proportions, can yield any other hue. Magenta is a mixture of red and blue; yellow is a mixture of green and red; and blue green (cyan) is a mixture of blue and green (Fig. 17).
![]() Fig. 17. Left. Colors that result when the primary additive colors are projected onto a screen. Right. Colors that result when the primary subtractive colored paints are mixed on a canvas. |
As mentioned previously, the sensitivities of the three cone pigments overlap (e.g., light of 540 nm and light of 590 nm stimulate both R and G receptors), yet we can easily distinguish between these two wavelengths (see Fig. 12). The light of 540 nm is green and that of 590 nm is yellow. The explanation lies in the relative stimulation of the three different kinds of cones and in the processing of the information by the retina and the brain.
It is believed that the hue center synthesizes information it receives from two intermediate centers: the R-G center and the B-Y center (Fig. 18). The information sent to the hue center from the R-G center depends on the relative stimulation of the R and G cones. For example, when light of 540 nm strikes the retina, it will stimulate both R and G cones (see Fig. 12). However, because the G cones are stimulated much more than the R cones, the message received by the hue center is predominantly green. On the other hand, if light of 590 nm strikes the retina, the R cones are stimulated more than the G cones, and we see yellow. When light of 630 nm strikes the retina, the G cones are not stimulated at all and we see red.
The B cones send information to the B-Y center. The Y information does not come from Y cones; there is no yellow-catching pigment. The deficit is corrected by the R and G cones, which send information that has the effect of yellow in the B-Y center.
Saturation (chroma) corresponds to the purity or richness of a color. When all the light seen by the eye is the same wavelength, we say that a color is fully saturated. As more wavelengths (or white light) are added, the eye still sees the same dominant hue, but the color is paler (desaturated). For example, pink is a desaturated red (Demonstration 2). Actually, all hues appear to change color as they are desaturated except yellow. See Abney effect, discussed later.
Brightness (also called luminance or value) refers to the quantity of light coming from an object (the number of photons striking the eye). Brightness is our subjective interpretation of luminance. If we place a filter over a projector and gradually (with a rheostat) lower its intensity, the brightness decreases.
To put some order into the naming of colors, Munsell arranged all perceptible colors in terms of hue, saturation, and brightness. Munsell charts allow us to refer to a given color by a number rather than by words like purple, maroon, plum, or magenta.
The reader may have noticed that brown was not represented on the color wheel or in the spectrum. This is because brown is a special type of color. It is perceived when we see a yellow or yellow red of low luminance or when a yellow red is presented within a white light of high intensity (Demonstration 3).
FACTORS THAT MODIFY COLOR PERCEPTION
BRIGHTNESS (BEZOLD-BRÜCKE PHENOMENON)
As brightness increases, most hues appear to change. At low intensities, blue green, green, and yellow green appear greener than they do at high intensities, which makes them appear bluer. At low intensities, reds and oranges appear redder, and at high intensities, they appear yellower (Demonstration 4). The exceptions are a blue of about 478 nm, a green of about 503 nm, and a yellow of about 578 nm. These are the wavelengths of invariant hue.
SATURATION (ABNEY EFFECT)
As white is added to a hue (desaturating it), the hue appears to change slightly in color. The effect is similar to adding yellow. Blue greens become greener and yellow greens become yellower. Reds and oranges also become yellower. The exception is a yellow of 570 nm (Demonstration 5).
STATE OF DARK ADAPTATION (PURKINJE EFFECT)
The relative luminosity curve illustrates the eye’s sensitivity to different wavelengths of light (Fig. 19). It is constructed by asking an observer to increase the luminance of lights of various wavelengths until they appear to be equal in apparent brightness to a yellow light whose luminance is fixed. When the eye is light adapted (daytime), yellow, yellow green, and orange appear brighter than do blues, greens, and reds. The cones’ peak sensitivity is to light of 555 nm.
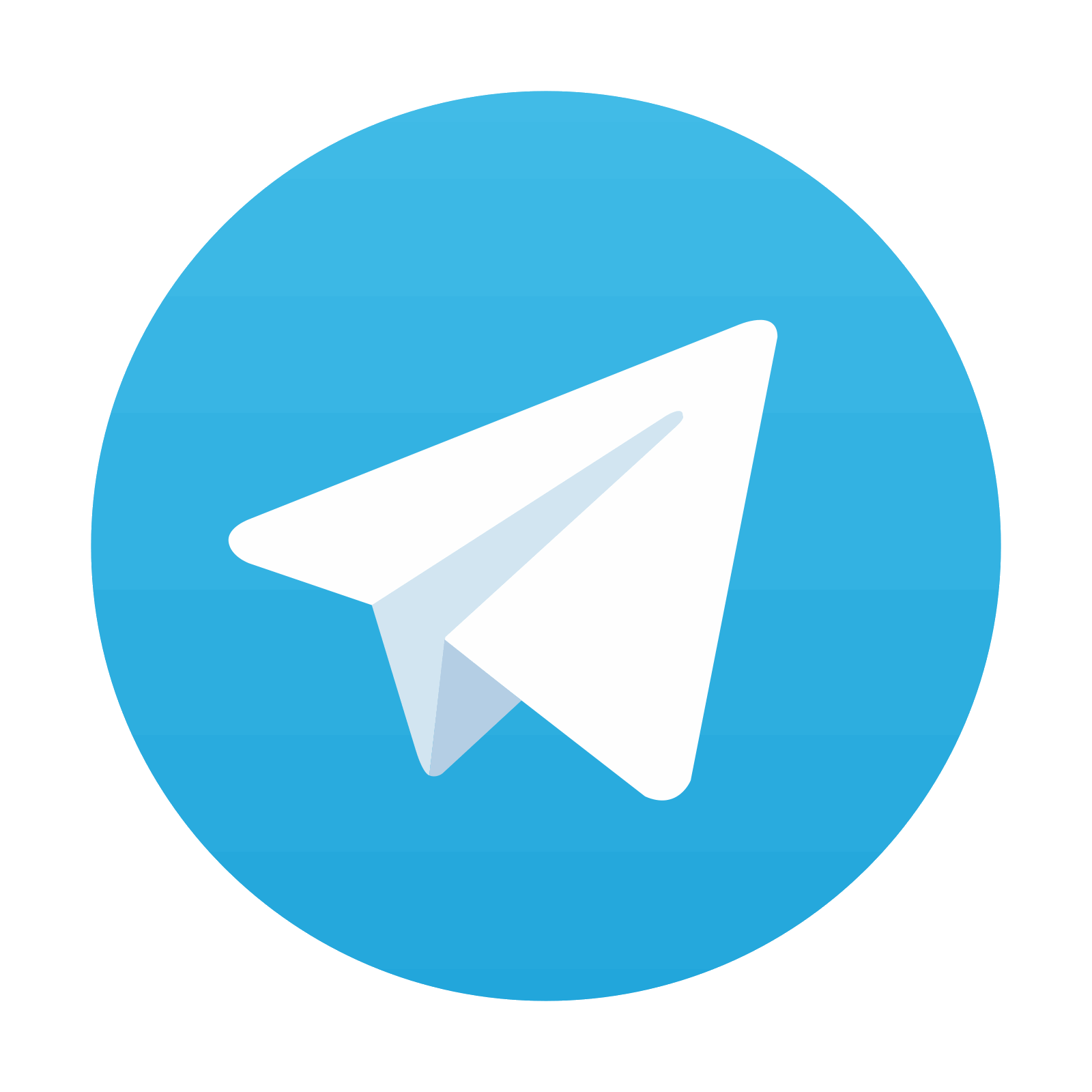
Stay updated, free articles. Join our Telegram channel
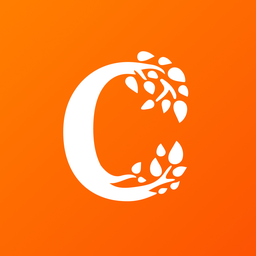
Full access? Get Clinical Tree
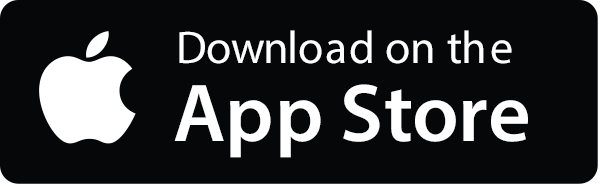
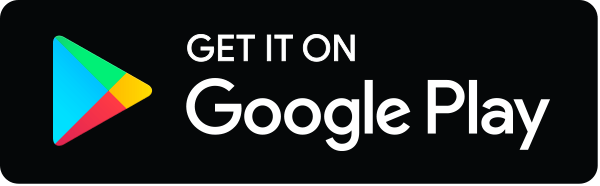