3Vestibular Physiology
Joel A. Goebel and Baran Sumer
The vestibular portion of the inner ear is anatomically suited for two main functions: stabilization of gaze during rapid impulsive head movements and postural control in a gravitational field. To function optimally, however, labyrinthine input is combined with visual, proprioceptive, auditory, and other sensory cues within the brainstem so that resultant eye and body movements are in context with the task at hand (Fig. 3–1). This sensory integration is the crucial element in gaze stabilization and postural control, and the vestibular end organ serves as an internal reference frame against which all other inputs are compared.
This chapter discusses basic physiology of the semicircular canals, utricle, and saccule in isolation, and explores fundamental elements of visual and proprioceptive mechanisms and how they interact with labyrinthine inputs. Central adaptation of the vestibulo-ocular reflex (VOR) to injury will be discussed, followed by case illustrations involving labyrinthine injury, abnormal visual or proprioceptive input, and sensory conflict.
Vestibular End-Organ Physiology
The basic sensory element of the vestibular end organ for the semicircular canals and the otolithic organs is the hair cell. Hair cells are specialized sensory cells that maintain a resting transmembrane potential of about -60 mV via selective permeability to cations, predominantly potassium. Each hair cell has an apical ciliary bundle made up of a single large kinocilium and multiple smaller stereocilia. The stereocilia are bound to the kinocilium of each hair cell, and all move together as a unit. When a force is applied parallel to the top of the cell deflecting the stereocilia toward the kinocilium, the hair cell is depolarized to -40 mV. Deflection of the stereocilia away from the kinocilium hyperpolarizes the cell to -64 mV. Forces perpendicular to the kinocilium-stereocilia axis have minimal effect on transmembrane potential1 (Fig. 3–2).
Figure 3–1 The multisensory nature of ocular motor and postural control. Sensory inputs from multiple sources are coded and compared within the brainstem, and modified by cortical and cerebellar connections, and the appropriate motor output is sent to the ocular muscles for gaze stabilization and to the antigravity muscles for postural stability. Feedback occurs to allow adaptation in the event of suboptimal ocular and postural control.
Figure 3–2 Example of a type I hair cell. The cell has a baseline firing rate at rest (A), which increases with deflection of the stereocilia (Sc) toward the kinocilium (Kc) and depolarization of the cell (B). Deflection of the stereocilia away from the kinocilium causes hyperpolarization and a decrease in the rate of neural firing (C). (Courtesy of Kamran Barin, Ph.D.)
There are two morphologically distinct types of hair cells. Type 1 hair cells have cell bodies that are globular in shape and are surrounded by calyceal nerve endings. Type 1 hair cells are concentrated toward the center of vestibular neuroepithelial structures, near the apex of the canal cristae and the striola of the otolith maculae. Their afferents have predominantly thick axons with fast conduction velocities and irregular firing rates that adapt to acceleration stimuli. Type II hair cells are columnar in shape and are contacted directly by multiple bouton synaptic endings. They are concentrated more on the periphery of the vestibular neuroepithelium. The afferents of type II hair cells tend to have thin, more slowly conducting axons with regular firing rates that tend to accurately encode prolonged acceleration stimuli. Both cell types also receive efferent synaptic contact.2
Semicircular Canals
Sensory Transduction
In the semicircular canals, hair cells are arranged along the crista ampullaris within the ampulla of each canal in a polarized fashion. Their cilia are embedded in the substance of the cupula, a neutrally buoyant gelatinous structure that spans the width and height of the ampulla. Angular head acceleration causes relative endolymph flow in a direction opposite to that of the head movement, which displaces the cupula. Cupular displacement in turn causes bending of the hair cell bundle, resulting in a change in membrane potential. Due to hair cell orientation on the cristae of the horizontal canals, ampullopetal flow (toward the ampulla) causes hair cell depolarization, whereas ampullofugal flow (away from the ampulla) causes hyperpolarization. In the posterior and superior canals, the hair cells have an opposite orientation, and this pattern of excitation and inhibition is reversed (Fig. 3–3).
Modeling of Semicircular Canal Dynamics
Endolymph flow and cupular displacement in the semicircular canals can be described mathematically using an inverted pendulum model. Simple mechanical considerations reveal that the inertial force of the endolymph and cupula and the viscous force of the endolymph will cause a time delay between the onset of an acceleration stimulus and maximal deflection of the cupula. In addition, the elastic force of the cupula acting against the viscous force will tend to return the cupula to its resting position when acceleration ceases. Both the initial displacement and the return of the cupula to its resting position will occur with an exponential time course.
Using an inverted pendulum model, one can predict an exponential time constant for an acceleration stimulus to produce initial cupular deflection. In humans, this time constant has a value of approximately 3 ms. The duration of most natural human head movements exceeds this interval, and inertial forces are important only for the briefest of head accelerations.3
According to the inverted pendulum model of cupular deflection, the cupula will return toward its resting position (and hair cells will repolarize) following the end of angular acceleration with a time constant of approximately 7 seconds. That is, it takes approximately 7 seconds for the cupula to return to within 37% of its original resting position. After four time constants, the return will be nearly complete. Experimental data, however, indicate that the time course of exponential decay of the slow phase velocity of nystagmus in response to step velocity rotations is consistent with a time constant of approximately 12 to 15 seconds, about twice as long as necessary for the return of the cupula to a resting position. This difference is accounted for by central nervous system perseveration of the vestibular afferent signal, a phenomenon that has been called velocity storage. It is believed to involve commissural connections between the vestibular nuclei and cerebellar projections. The system is charged by head acceleration and discharges over an interval after the acceleration has ceased. The main purpose of velocity storage appears to be to perseverate low-frequency (low-acceleration) vestibular input, effectively extending the dynamic range of the system.4
Figure 3–3 Orientation of semicircular canals. The lateral semicircular canals lie in the same horizontal plane. The right superior (anterior) canal lies parallel to the left posterior canal and the left superior (anterior) canal lies parallel to the right posterior canal. LSCC, lateral semicircular canal; ASCC, anterior (superior) semicircular canal; PSCC, posterior semicircular canal. (Courtesy of Kamran Barin, Ph.D.)
During sinusoidal head rotation, time constants derived from the model above can be used to predict the frequency response of the gain or strength of the reflex, and phase or timing. For very low frequency rotations (below 0.1 Hz), the rotational stimulus must overcome the elastic restoring force of the cupula. Gain is directly related to acceleration (which is proportional to frequency) and phase is less than zero—eye velocity “leads” head velocity. For rotations between approximately 0.1 and 5 Hz, the restoring forces are easily overcome. Gain is near 1 (perfectly compensatory) and phase is nearly 0. Over this range, the canals act as velocity integrators so that eye velocity is directly compensatory to head velocity. However, at frequencies above 5 Hz, the effects of the short time constant begin to dominate as the brevity of acceleration for each half cycle fails to maximally deflect the cupula before the onset of the counterrotational acceleration of the next half cycle. At these higher frequencies, gain declines and phase becomes greater than zero—eye velocity “lags” head velocity.
Primary Canal Afferents
All vestibular afferent neurons have a resting discharge rate of approximately 90 spikes per second (range: 10 to 200 spikes/s). This resting discharge is required for bidirectional sensitivity. Excitatory canal rotation produces depolarization of hair cells, which leads to an increase in the neuronal firing rate. Rotation in the opposite direction causes hair cell hyperpolarization and leads to a decrease in the firing rate. For low acceleration rotational stimuli, the modulation of firing rate around an individual neuron’s mean is virtually symmetrical. At higher acceleration, however, the firing rate becomes increasingly asymmetrical because upward modulation is not limited, whereas downward modulation cannot go below zero.5
The transduction of head rotation in the yaw plane in the semicircular canals is graphically represented in Fig. 3–4. Leftward head rotation (indicated by the bold arrow) induces relative endolymph flow to the right (thin arrows) due to inertial forces of the fluid. This in turn causes ampullopetal cupular displacement in the left horizontal canal and ampullofugal displacement in the right horizontal canal. Hair cells in the crista of the left canal are depolarized, and those in the right canal are hyperpolarized. This causes an increase in the neuronal firing rate in the left vestibular nerve and a decrease in the neuronal firing rate in the right. Although the strongest input is provided by the ipsilateral canal (i.e., the canal on the side toward which the end is turned), this “push-pull” mechanism amplifies the asymmetric neural input to the vestibular nuclei.
Otolith Organs
Sensory Transduction
Otolith hair cell cilia are embedded in the gelatin layer of the otolithic membrane of the utricle and saccule that underlies the layer of otoconia (composed primarily of calcium carbonate crystals). Because the otoconia have a higher specific gravity than endolymph, their greater inertia causes them to be displaced upon the hair cell layer by applied linear acceleration (including gravity) in the plane of the otolithic membrane. As with all hair cells, maximal activation occurs along a vector deflecting the stereocilia toward the kinocilium. Compressive forces have no effect. Acceleration due to gravity exerts a constant shearing force on all portions of the macula except those perfectly aligned to the earth-horizontal plane. Hair cell activation can be changed by a static head tilt, which alters the orientation of the maculae with respect to the gravitational vector, or by translational movements of the head without change in head orientation, or a combination of both.6
Figure 3–4 Binaural response of the horizontal canals to leftward head rotation in the yaw plane. The left canal hair cells depolarize, thus increasing the neural firing rate over the baseline activity. Conversely, hyperpolarization of the right canal hair cells causes a decrease in neural activity. Taken together, these two changes enhance the difference in signals to the brainstem that code rotation to the left.
The organ of sensory transduction in the otoliths consists of the maculae of the utricle and the saccule. The hair cells of both otolith organs are oriented at right angles to a central curvilinear axis called the striola. Oppositely oriented hair cells are separated by the striola, giving the maculae bidirectional sensitivity. The utricular macula is oriented roughly perpendicular to the earth-vertical plane in normal head position (i.e., roughly in the same plane as the horizontal semicircular canal), and the saccular macula roughly parallel to the earth-vertical plane. There are minimum and maximum stimuli to which the otoliths may respond. Threshold forces are approximately 1/20 of the acceleration of gravity (0.05g). Otolith responses are linear up to approximately 1g and saturate above this point (Fig. 3–5).7
Primary Otolith Afferents
Like semicircular canal afferents, otolith afferent neurons have a resting firing rate that is modulated up or down depending on hair cell activation by linear acceleration or gravity. Baseline neuronal activity is measured when hair cells are oriented parallel to the gravitational vector (and the otolithic membrane is oriented perpendicular to it) when no shear forces are present. This is also the orientation in which the hair cells have the greatest sensitivity to linear acceleration. Otolith afferent activity is modulated upward or downward depending on the combination of orientation by static head tilt, which alters the orientation of the maculae with respect to the gravitational vector, and translational movements of the head, which create transient linear forces. The firing rate of a particular neuron may not directly correlate with individual hair cell activation as in the canal afferents because otolith afferents collateralize to receive input from several hair cells, which may not be coplanar.8
Vestibular Efferents
Efferent neurons also are present in the semicircular canals and otoliths. Evidence exists that vestibular efferents modulate afferent neuron sensitivity or firing rates, but their exact function is far from clear. Further information on the hypothesized substrate and function of this system can be found elsewhere.2,9
Vestibulo-Ocular Reflex
The purpose of the VOR is to stabilize images on the retina particularly during rapid impulsive head movements. These movements can be highly variable in frequency and velocity, such as voluntary changes in head position, subtle perturbations due to footfalls during locomotion, or transmitted effects of the cardiac pulse. The VOR is perhaps the best studied of all central reflexes because its stimulus transduction and central connections are relatively well understood, and its output is relatively easily measured. It is also an important diagnostic tool because by analyzing the reflex we can indirectly assess vestibular function.
Figure 3–5 Orientation of the otolith organs. The utricle is oriented roughly perpendicular to gravity and the saccule lies parallel to gravity when the subject is upright. Arrows indicate orientation of hair cells and direction of maximal stimulation relative to the striola. (Courtesy of Kamran Barin, Ph.D.)
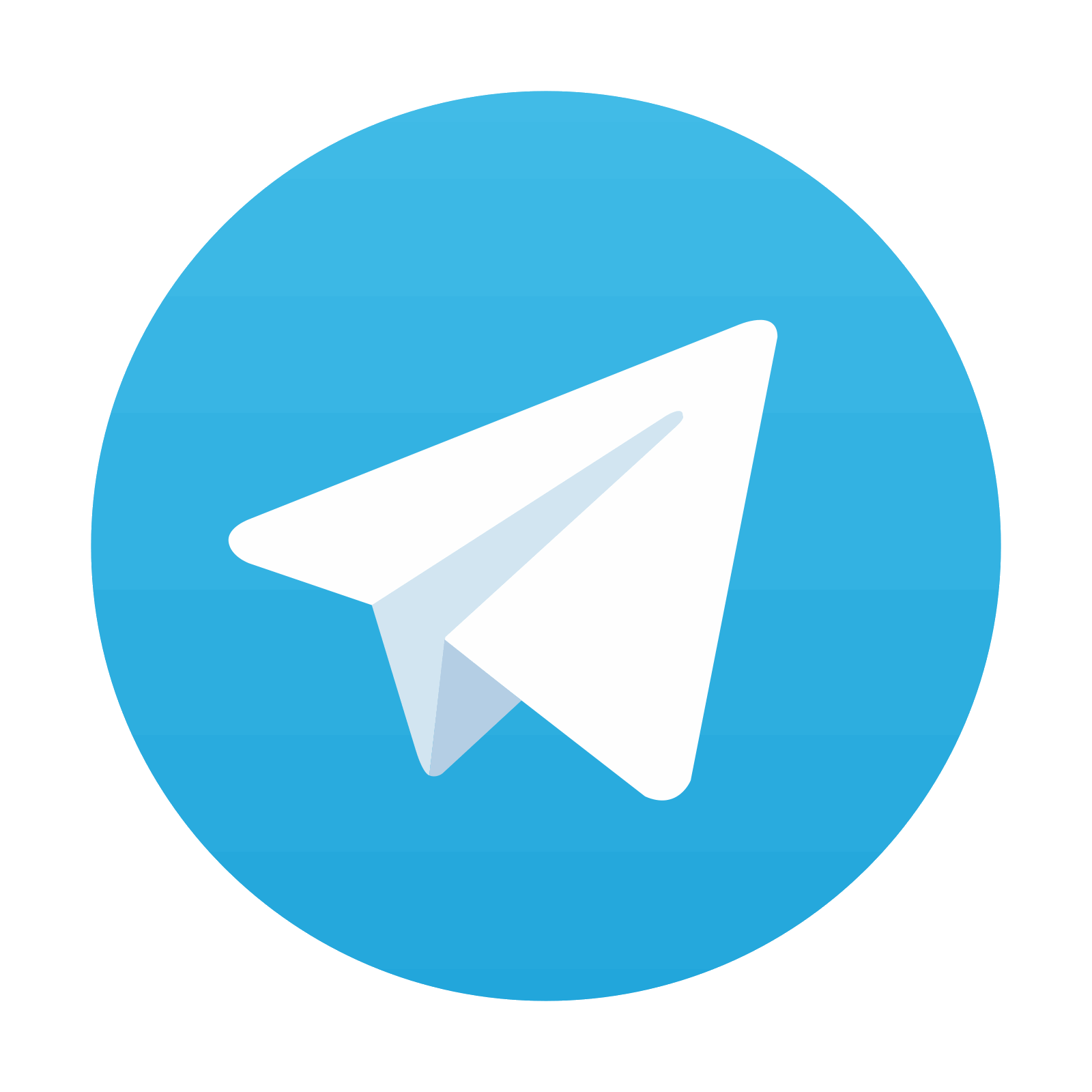
Stay updated, free articles. Join our Telegram channel
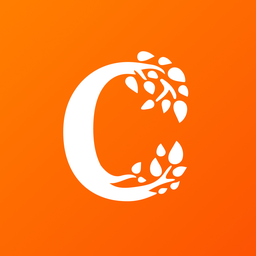
Full access? Get Clinical Tree
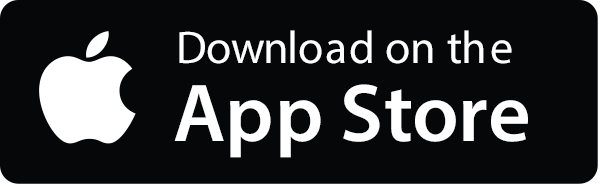
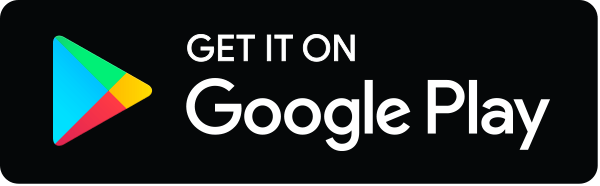