Structure and Function of the Auditory and Vestibular Systems
Hearing is a complex process that is critical to our everyday functioning. As humans, we can hear an extremely wide range of frequencies and intensities. We can also discriminate small changes in intensity (on the order of 1 dB) and frequency (on the order of a few hertz). Even more amazing is the fact that the normal human auditory system can detect sounds embedded in noise and process what someone is saying from across the room at large social gatherings (i.e., the cocktail party effect). These hearing skills, which we all take for granted, are a result of the ear (peripheral system) and the brain (central system) working together harmoniously with great precision.
The vestibular system, which shares some anatomic structures with the auditory system, is responsible for our ability to perceive changes in head movements (acceleration and deceleration) and the orientation of the head with respect to gravity. Similar to the auditory system, the vestibular system has both peripheral as well as central components that work together to help us maintain our sense of balance.
Understanding the structure and function of the peripheral and central components of these two systems is the goal of both basic and clinical research. It also is critical for advances in the diagnosis and treatment of disorders of the auditory system and any comorbid or related vestibular problems. An appreciation and understanding of the anatomy and physiology of these systems allows one to identify the location of the disordered region within the auditory and vestibular systems. This in turn provides insight as to the nature of the disorder that can lead to effective treatment. Therefore, it is safe to say that understanding disorders of the auditory and vestibular systems is an essential skill for the audiologist and hearing care professional that is dependent on a substantial knowledge of the structure and function of these two interrelated systems.
Prior to a detailed discussion of anatomy and physiology, it is important for the reader to have a fundamental understanding of the basic terminology used to describe the location and orientation of anatomic structures. Knowledge of this terminology provides the clinician with the ability to discuss anatomic position, eliminate ambiguity, and reduce medical errors. For example, when discussing a tympanic membrane perforation, if appropriate anatomic terminology is used, a clear distinction can be made indicating whether the location of the perforation is anterior or posterior and inferior or superior. These terms, as well as other common anatomic terms, will be used throughout this book. Table 2–1 provides the reader with a list of the more commonly used anatomic terminology along with a brief definition of each term.
THE PERIPHERAL AUDITORY SYSTEM
The temporal bone either houses or supports most of the structures of the auditory periphery. It is an integral part of the skull base and is composed of four fairly distinct segments: the squamous, the tympanic (bony ear canal), the mastoid, and the petrous segments. The squamous portion is part of the lateral cranium immediately superior to the ear canal. The bony ear canal is another segment of the temporal bone and constitutes the tympanic portion. Directly posterior to the tympanic portion is the mastoid segment, which is characterized by numerous air cells. The final segment is the petrous portion that houses the middle ear, the cochlea, and the vestibular apparatus. It is a wedge-shaped structure that courses medially in the base of the skull and divides the posterior cranial fossa from the middle cranial fossa (Figure 2–1).
On the posterior side of the petrous portion of the temporal bone there are a number of key structures. The opening to the internal auditory meatus (IAM), also termed the porous acusticus, is located about two-thirds of the way along this structure and courses in a lateral to medial direction. Through this opening in the temporal bone exits the auditory, vestibular, and facial nerves that project to their respective nuclei in the brainstem (lateral, caudal pons). Also located on the posterior aspect of the petrous bone just lateral to the IAM opening is the opening for the vestibular and cochlear aqueducts. These openings are identified by small recesses in the posterior side of the petrous bone (Anson & Donaldson, 1981).
Figure 2–1. A photograph of the base of the cranium with anterior, posterior, and lateral aspects depicted. Key: PF = posterior fossa, MF = middle fossa, FM = foramen magnum, p = petrous portion of the temporal bone which houses the middle and inner ears as well as the internal auditory meatus. From The Auditory System: Anatomy, Physiology, and Clinical Correlates (2nd edition, p. 88), by F. E. Musiek and J. A. Baran, 2020, San Diego, CA: Plural Publishing. Copyright © 2020 Plural Publishing, Inc. All rights reserved.
One of the main functions of the temporal bone is to provide a framework of support for the outer, middle, and inner ears, as well as the seventh and eighth cranial nerves. In addition to support and stabilization of these structures, the temporal bone provides protection for most of the anatomic structures within the auditory periphery that support hearing.
Structure
The outer or external ear includes the pinna (also referred to as the auricle) and the external auditory meatus (EAM) or ear canal. The pinna is C shaped and composed of a foundation of cartilage that is covered with skin. The structure of the pinna takes on a shape that conforms to the underlying cartilage, which results in its distinct appearance as a structure with numerous folds and recesses. These folds and recesses constitute specific anatomic sites or areas within the pinna for which particular terms are used (Figure 2–2). Referring back to the C-shaped structure of the pinna, the outer circular part of the C is termed the helix and just inside of the helix is the antihelix. Located superiorly between the helix and antihelix is a groove called the scaphoid fossa, which lies next to the triangular fossa. Immediately inferior to the antihelix is a ridge termed the crus of the helix. This ridge is responsible for much of the rigidity of the pinna. The deepest recess of the pinna is the concha, which leads to the opening of the ear canal. Anterior to the concha is a protective flap called the tragus, which can be pressed into the opening of the ear canal to serve as protection. Inferior and posterior to the tragus is a prominence termed the antitragus. At the most inferior aspect of the pinna is the ear lobe, probably the softest and most flexible of the pinna’s structures. Between the ear lobe and the tragus is the intertragal incisure (Clark & Ohlemiller, 2008; Musiek & Baran, 2020). The various structures of the pinna are innervated by the fifth (trigeminal) and seventh (facial) cranial nerves (Musiek & Baran, 2020; Zemlin, 1998).
Figure 2–2. A photo of a human right pinna. From The Auditory System: Anatomy, Physiology, and Clinical Correlates (2nd edition, p. 46), by F. E. Musiek and J. A. Baran, 2020, San Diego, CA: Plural Publishing. Copyright © 2020 Plural Publishing, Inc. All rights reserved.
The anatomy of the pinna becomes useful when taking earmold impressions and when fabricating earmolds. Variances in the pinna’s fine structure can be well defined using the anatomy discussed previously. This in turn can allow better and more precise communication between the audiologist and the earmold manufacturer concerning the need for attention to a particular area or areas of the earmold to be created or modified, which should result in a better fit, and ultimately, a more satisfactory hearing aid experience for the patient.
The second structure in the external ear is the EAM. This outer ear structure is shaped like a tube and averages 2.5 cm to 3.0 cm in length and 0.75 cm in diameter in the adult human. Obviously, these dimensions would be much smaller in pediatric patients. The EAM originates at the concha of the pinna and ends at the tympanic membrane (Figure 2–3). The EAM is not straight, but rather curves like an elongated letter S that is lying on its side. However, it is important to note that there is great variation in the shape of the EAM in humans. Visual inspection of this structure will reveal some EAMs that are relatively straight, whereas in other instances, the EAM will be observed to be curvier or more tortuous. Pulling on the posterior aspect of the pinna can help straighten the ear canal for visual inspection with otoscopy and allow for a better seal during tympanometry and acoustic reflex testing. This procedure can prove to be especially helpful when one is visually inspecting an ear canal that is quite curvy in nature.
The foundation of the outer third of the ear canal is cartilaginous, whereas the foundation of the medial two-thirds is bony. The entire length of the ear canal is covered by an epidermal lining and the outer third contains hair follicles and glands that secrete a waxy substance resulting in the production of cerumen. Innervation of the EAM comes from the fifth (trigeminal), seventh (facial), and ninth (glossopharyngeal) cranial nerves, making the EAM sensitive to tactile stimulation (Møller, 2000; Musiek & Baran, 2020; Zemlin, 1998).
Function
The pinna’s main function is to help funnel sounds from the environment into the smaller diameter EAM. Because of its unique structure and size, the pinna tends to result in a slight enhancement of sounds in the vicinity of 5000 Hz (Shaw & Teranishi, 1968). This enhancement occurs because the unique configuration of the pinna in terms of its ridges and recesses results in a more efficient collection of sound in the higher frequency range, whereas the wavelengths of lower frequency sounds are essentially larger than the pinna and can pass around this structure more readily than those of higher frequency sounds (Musiek & Baran, 2020). The pinna also helps with sound localization by creating complex resonances that change as the location of the sound source changes (Blauert, 1983).
Figure 2–3. A drawing of the outer, middle, and inner ears showing the location and configuration of the external auditory meatus. Key: EAM = external auditory meatus, ME = middle ear, CO = cochlea. Adapted with permission from The Auditory System: Anatomy, Physiology, and Clinical Correlates (2nd edition, p. 1), by F. E. Musiek and J. A. Baran, 2020, Copyright © 2020 Plural Publishing, Inc. All rights reserved.
The EAM helps to protect the ear with its debris-catching cilia and cerumen, and it also serves as an acoustic resonator. The EAM peak resonance is around 3000 to 4000 Hz in the adult. At these frequencies, there is a gain of 10 to 15 dB in the acoustic signal with little or no gain at the frequencies below 1000 Hz (Dallos, 1973).
It also should be mentioned that in addition to the pinna and the EAM, the head and even the torso can exert differential effects on the sounds reaching the ear. The combination of these effects provides valuable auditory information that the normal auditory system uses to help identify the source of a sound (i.e., directional hearing). Most notable of the directional hearing effects is the head shadow effect, which can have a significant effect on the sound reaching the ear when the sound is originating from a source located on one side of the head versus the other. Take, for example, the situation where one has a sound being presented in a sound field to the right side of the head. In this case, the sound would have almost direct access to the right ear, but the head would interfere with the sound that is traveling to the left ear. The end result would be that the sound reaching the left ear would be of a lower intensity (especially at high frequencies) than the sound arriving at the right ear. The central auditory system is capable of detecting and analyzing these types of intensity differences, as well as the “small” time of arrival differences that occur as a result of the further distance that the signal has to travel to reach one ear versus the other in order to accurately identify the source of a sound (for additional discussion of directional hearing effects, see Musiek & Baran, 2020).
Structure
The tympanic membrane (TM) marks the beginning of the middle ear and is oval shaped and concave in its appearance (Figure 2–4). In the adult human, it averages 8 to 10 mm in diameter, with a slightly larger diameter along the vertical axis than along the horizontal axis. The membrane has three layers and measures about 0.1 mm in thickness (Gelfand, 1997; Zemlin, 1998). These three layers include the epidermal (outer), the fibrous (middle), and the membranous (mucosal lining) layers. The fibrous layer is thicker in the center portion of the TM and thinner in the superior portion of this membrane. This “thinner” area is called the pars flaccida, which is the most elastic portion of the TM. For the most part, the remainder of the TM is stiffer (containing more fibers) and is referred to as the pars tensa (see Yost, 2000).
Figure 2–4. An illustration of the tympanic membrane superimposed on the middle ear ossicles and related ear structures observed from this lateral view. Adapted by Frank E. Musiek and Jane A. Baran in The Auditory System: Anatomy, Physiology, and Clinical Correlates, 2020. Original from The Human Ear in Anatomical Transparencies by Stephen Polyak, Gladys McHugh, and Delbert Judd, 1946.
The TM has several important anatomic landmarks in addition to the pars flaccida and the pars tensa. The annular ligament is the rim around the TM, which anchors the membrane to the wall of the ear canal. The manubrium of the malleus attaches to the TM in its upper center portion, and the umbo is located in the center of the TM and marks the point of attachment for the most lateral aspect of the malleus (see Figure 2–4). Coursing inferiorly and in a slightly lateral direction is the cone of light (usually at ~5 o’clock for the right TM and ~7 o’clock for the left TM), which simply is the light of the otoscope that is reflected off the TM during otoscopy. With good observation skills and a translucent tympanic membrane, it is possible to visualize the long process of the incus through the TM. If observed, the location of the long process would be viewed in the superior-posterior quadrant of the TM.
The middle ear is identified, in part, by an air-filled cavity within the temporal bone that is bordered by the bony capsule of the cochlea medially and aspects of the temporal bone superiorly (attic), anteriorly, and posteriorly. The medial wall of the cochlea has two openings: the oval window (superior) and the round window (inferior), which are separated by the promontory. The ossicular chain, perhaps the most obvious structure in the middle ear, is composed of three bones: the malleus, the incus, and the stapes. Each of these bones has a detailed anatomy (see Musiek & Baran, 2020, for review). The stapes, the final bone in the ossicular chain, covers the oval window, and the round window is covered by a flexible membrane (also known as a secondary tympanic membrane) (Musiek & Baran, 2020; Zemlin, 1998).
In the anterior, inferior middle ear cavity is the opening to the Eustachian tube. This tube connects the middle ear cavity with the posterior aspect of the nasopharynx. Also located in the middle ear is the stapedius muscle tendon, which arises from the posterior wall of the middle ear and connects to the head of the stapes, as well as the tendon of the tensor tympani muscle, which courses through the middle ear cavity to connect to the malleus. In addition, a branch of the facial that innervates the stapedius muscle (the smallest muscle in the body) transverses the middle ear space.
Function
Due to its concave structure, the TM has a rather complex displacement pattern that accommodates a wide range of frequencies and intensities (see Zemlin, 1998, for more in-depth explanation). Maximum displacement of the TM occurs in different areas and is dependent on the frequency of the sound stimulus. At high frequencies, the TM tends to vibrate in segments, whereas at low frequencies, there is less segmental vibration and the TM tends to vibrate more as a single unit (Gelfand, 1998).
A variety of functions are associated with the middle ear. The middle ear is an air-filled cavity. The volume of air within this cavity acts as a filter that limits or alters the transmission of some low-frequency sounds through the system. The structure also contributes to some complex interactions between the ear canal and pinna resonances, which alter the transmission of sound across the frequency range. The end result is primarily an increase in the intensity of the high-frequency components reaching the cochlea when these are compared to the levels represented in the original signal arriving at the outer ear.
The ossicular chain transmits vibrations from the eardrum to the cochlea. The stapes, the final bone in the ossicular chain, transmits these vibrations to the cochlea by horizontally rotating around an axis (a rockinglike movement) at high intensities, whereas a more pistonlike movement of the stapes occurs for low intensities. The former type of movement may be related to stapedius muscle contraction, which occurs at high intensities (see Musiek & Baran, 2020, and Gelfand, 1998, for details). The structure of the ossicular chain and its ligaments serve to dampen high intensity sounds and stabilize the middle ear transmission process (Musiek & Baran, 2020).
The Eustachian tube’s primary function is to allow fresh air into the middle ear cavity and balance the air pressure in the middle ear to that of the atmosphere. This is done when one opens the mouth or swallows. These actions result in contractions of the tensor veli palatini and levator veli palatini muscles, which function to open the Eustachian tube (Zemlin, 1998). The Eustachian tube also protects the middle ear from fluid or solids arising from the nose and/or mouth as this structure remains closed at rest.
Transformer Action. Sound travels readily through air, which is a low impedance medium. However, in hearing, sound (i.e., changes in air pressure) must be directed to a fluid-filled system, the cochlea, where the impedance is quite high. Therefore, without some help from the transformer action of the middle ear, most of the acoustic energy reaching the cochlea would be reflected back out of the ear. Three mechanisms contribute to the middle ear transformer effects: (1) an area ratio advantage between the TM and the stapes’ footplate, (2) a lever advantage created by the middle ear bones, and (3) a “buckling” advantage due to the concave structure of the TM. The area ratio advantage relates to the fact that the TM has a much greater area than the stapes’ footplate, which is the point of energy transfer to the inner ear. The area ratio of the TM to the stapes’ footplate is about 22:1, but the pars flaccida of the TM likely contributes little to this area ratio; hence, the effective ratio is estimated to be about 17:1. This area ratio focuses energy at the stapes’ footplate, thus increasing the input greatly at this point in the auditory system (Gelfand, 1998; von Békésy, 1960). The lever advantage is created by the way the malleus and incus interact. The gain in force is related to the longer handle of the malleus (manubrium) moving the shorter handle of the incus (long process) for about a 1.3:1 ratio advantage. Finally, the buckling action relates to the inward curvature of the TM, which on vibration imparts energy to the malleus. When this happens, the TM moves proportionately more than the malleus (i.e., a “buckling effect”). The smaller displacement at the malleus in reference to the TM creates a greater force or a gain in force (Musiek & Baran, 2020). These three transformer mechanisms allow greater energy to be directed to the fluid-filled cochlea than would be the case if these mechanisms did not exist or did not function appropriately. If these mechanisms are compromised individually or collectively, a conductive hearing loss is expected.
The Acoustic Reflex. Stapedius muscle contraction is the end point of the acoustic reflex (AR). Because the stapedius muscle is located in the middle ear, it is reasonable to discuss this reflex in this section of this chapter. However, it is important to note that the AR involves both peripheral and central mechanisms (Figure 2–5).
The AR pathway starts with sound entering the external ear and passing through the middle ear to the cochlea. The auditory nerve (see Figure 2–5) picks up the impulses from the cochlea and directs them to the ventral segment of the cochlear nucleus, which is located in the caudal brainstem. The ventral cochlear nucleus then sends a fiber tract directly to the ipsilateral facial nerve nuclei and another branch to the contralateral superior olivary complex. The cochlear nucleus also sends fibers to the ipsilateral superior olivary complex, which then connects to its contralateral counterpart. The ipsilateral superior olivary complex connects to the facial nerve nuclei on both sides, and the contralateral superior olive also has input to the facial nerve nuclei. Hence, there is bilateral input to the facial nerve nuclei resulting in bilateral acoustic reflexes, even if there is only monaural stimulation. From the facial nerve nuclei, the AR follows an efferent course back to the stapedius muscle in the middle ear (Borg, 1973).
Møller (2000) and Borg (1973) provide excellent accounts of the nature and physiology of the acoustic reflex. Probably the most popular explanation offered for the purpose of the acoustic reflex is that it serves to attenuate high-intensity sounds and therefore may help to protect the inner ear. As has been documented by Møller (2000), the higher the intensity of an acoustic signal above the reflex threshold intensity, the greater the contraction of the AR, at least for individuals with normal auditory and facial nerve function. The action of the AR could be viewed as contributing to the nonlinearity of intensity coding within the auditory system as it results in a compression effect. In humans with normal auditory function, the AR is initiated at 70 to 90 dB HL for tonal stimuli and frequencies from 250 to 4000 Hz have little or no differential effects on the level of the threshold of the AR that is measured. The amplitude of the AR is largest for bilateral stimulation followed by ipsilateral and then contralateral stimulation (see Musiek & Baran, 2020). The latency of the AR also is variable and depends on a host of factors including stimulus type, intensity level, frequency, and how it is recorded.
Structure: The Bony Cochlea
The cochlea is a bony shell similar in shape to a snail shell that is located within the petrous portion of the temporal bone (Figure 2–6). Inside this snaillike shell are fluids (endolymph and perilymph), special cells (hair and supporting cells), membranes (basilar, tectorial, Reissner’s), nerve fibers, blood vessels, and special epithelium. The cochlea makes up part of the inner ear that also includes the vestibular apparatus. The latter structure has a highly identifiable external bony structure that includes the three semicircular canals and the less obvious areas for the utricle and saccule. The bony cochlea has two openings in the shell. The more superior is the oval window, which articulates with the stapes, and inferior to the oval window is the round window. As mentioned earlier, a bony eminence between the two openings is called the promontory (Zemlin, 1998).
Figure 2–5. A schematic of the acoustic reflex with the acoustic stimulus presented to the left ear. The vertical dotted line in the middle of the drawing represents the midline of the head at the brainstem level. From The Auditory System: Anatomy, Physiology, and Clinical Correlates (2nd edition, p. 240), by F. E. Musiek and J. A. Baran, 2020, San Diego, CA: Plural Publishing. Copyright © 2020 Plural Publishing, Inc. All rights reserved.
Figure 2–6. A photo of the bony cochlea situated in the temporal bone. Key: 1 = basal turn, 2 = helicotrema. (Courtesy of M. Pinheiro.) From The Auditory System: Anatomy, Physiology, and Clinical Correlates (2nd edition, p. 5), by F. E. Musiek and J. A. Baran, 2020, San Diego, CA: Plural Publishing. Copyright © 2020 Plural Publishing, Inc. All rights reserved.
Inside the bony cochlear shell is a spiral-shaped osseous structure called the osseous spiral lamina (OSL). The OSL is shaped like an evergreen tree with the basal part of the cochlea located where the lower branches would be, and the apical part of the cochlea located toward the top of the tree (Figure 2–7). The bony cochlea is approximately 1 cm wide at the base and about 0.5 cm in length from base to apex. The human cochlea’s “spiral” has from 2.2 to 2.9 turns. The modiolus is the central part of the structure, which is composed of perforated bone that allows auditory nerve fibers to pass through small openings in the OSL and connect to the hair cells. The OSL has a shelf that spirals up the modiolus. This bony shelf is the supporting structure for the basilar membrane and the limbus. This shelf is wider at the base than at the apical end of the cochlea (Musiek & Baran, 2020; Zemlin, 1998).
Function: The Bony Cochlea
In general, the bony cochlea serves as a framework of support and protection for the membranous cochlea and the auditory nerve fibers. The oval and round windows supply access to the membranous inner ear and seal the inner ear fluids with the stapes and the round window membrane serving these functions. Because of its position, the promontory protects the round window. For example, if one were to perforate the lower segment of the TM with a probe, the probe would likely contact the promontory and not the round window. This is what happens when transtympanic electrocochleography is conducted. That is, after piercing the lower TM, a needle electrode comes into contact with the promontory where the electrophysiologic recording takes place.
Figure 2–7. A drawing of the osseous spiral lamina. The bony shelf spirals around the modiolus from base (bottom of figure) to apex (top of figure). From The Auditory System: Anatomy, Physiology, and Clinical Correlates (2nd edition, p. 6), by F. E. Musiek and J. A. Baran, 2020, San Diego, CA: Plural Publishing. Copyright © 2020 Plural Publishing, Inc. All rights reserved.
Structure: The Membranous Cochlea
The bony cochlea provides a spiral framework that is followed by the membranous cochlea (Figure 2–8). The membranous cochlea contains three ducts called scalae: the scala vestibuli (superior), the scala tympani (inferior), and the scala media—the smallest scala that is situated between the other scalae (often termed the cochlear duct) (Figure 2–9). The stapes’ footplate articulates with the oval window, which opens into the vestibule of the inner ear and transfers energy down the scala vestibuli. The round window is located at the end of the scala tympani. Reissner’s membrane and the basilar membrane (BM) divide the cochlea into the three ducts. Reissner’s membrane separates the scala vestibuli from the scala media, and the BM divides the scala media from the scala tympani. These ducts run the length of the cochlea except at the very end, termed the helicotrema, where the scala vestibuli and the scala tympani communicate. The scala media communicates with the vestibular system (specifically the saccule) via a narrowed channel called the ductus reuniens.
Two important fluid channels, the vestibular and cochlear aqueducts (VA, CA), play key roles in inner ear function. The VA is an endolymphatic channel that communicates from the posterior surface of the petrous bone to the saccule. The VA encompasses the endolymphatic duct and sac. The CA, which also opens to the petrous bone’s posterior surface, courses to the scala tympani near the round window. This channel contains perilymph, a fluid highly similar to cerebral spinal fluid. The two main fluids in the cochlea are endolymph (in the scala media) and perilymph (in the scala vestibuli and scala tympani). Endolymph is high in potassium and low in sodium, whereas perilymph has just the opposite chemical concentrations (Figure 2–10).
Figure 2–8. A drawing of the membranous labyrinth. Key: SSC = superior semicircular canal, LSC = lateral semicircular canal, PSC = posterior semicircular canal. From The Auditory System: Anatomy, Physiology, and Clinical Correlates (2nd edition, p. 96), by F. E. Musiek and J. A. Baran, 2020, San Diego, CA: Plural Publishing. Copyright © 2020 Plural Publishing, Inc. All rights reserved.
The BM is composed of about 24,000 fibers and is 25 to 35 mm in length in the adult human. The BM supports the organ of Corti, the actual end organ for hearing. The adult BM is wider at its apex than at its base (0.36–0.5 mm versus 0.04–0.1 mm), and it is both thicker and stiffer at the base than at the apex (Buser & Imbert, 1992; Musiek & Baran, 2020) (Figure 2–11). Reissner’s membrane is essentially avascular, has two layers of cells, and is permeable. From base to apex, Reissner’s membrane becomes wider like the BM; however, the scala media and the “shelf” of the spiral lamina become smaller and narrower, respectively, as one moves from the base to the apex.
The organ of Corti is composed of supporting structures, sensory cells, membranes, and nerve fibers (Figure 2–12). This structure courses the length of the cochlea. Directly superior to the hair cells is the tectorial membrane, which interacts with the stereocilia of the outer hair cells and is anchored to the upper lip of the limbus. It appears that the tectorial membrane does not articulate with the stereocilia of the inner hair cells. On its underside and just above the inner hair cells’ stereocilia is a bulge termed Hensen’s stripe. This bulge in the membrane’s underside reduces the distance between the tops of the stereocilia and the tectorial membrane and may play a role in stimulation of the inner hair cells (see discussion later).
Figure 2–9. A drawing of a cross-section of the cochlea showing the three scalae. Key: OHC = outer hair cells, IHC = inner hair cell. From The Auditory System: Anatomy, Physiology, and Clinical Correlates (2nd edition, p. 97), by F. E. Musiek and J. A. Baran, 2020, San Diego, CA: Plural Publishing. Copyright © 2020 Plural Publishing, Inc. All rights reserved.
The reticular lamina is composed of tightly packed cells and forms a ceiling above the sensory and supporting cells of the organ of Corti (Figures 2–12 and 2–13).
Figure 2–10. A sketch of the membranous inner ear with a focus on the cochlear and vestibular aqueducts. Key: A = endolymphatic sac, B = vestibular aqueduct, C = endolymphatic duct, D = saccule, E = utricle, F = semicircular canals, G = ductus reunions, H = cochlear aqueduct, I = round window, J = stapes (oval window), K = scala vestibuli, L = scala media, M = scala tympani. From The Auditory System: Anatomy, Physiology, and Clinical Correlates (2nd edition, p. 97), by F. E. Musiek and J. A. Baran, 2020, San Diego, CA: Plural Publishing. Copyright © 2020 Plural Publishing, Inc. All rights reserved.
Figure 2–11. A drawing looking down on the basilar membrane showing the relative width and stiffness dimensions of the basilar membrane from its apical to basal end (A), and the relationships between the basilar membrane and the bony shelf of the cochlea from apex to base (B). Adapted from The Auditory System: Anatomy, Physiology, and Clinical Correlates (p. 85), by F. E. Musiek and J. A. Baran, 2016, San Diego, CA: Plural Publishing. Copyright © 2016 Plural Publishing, Inc. All rights reserved.
Figure 2–12. A drawing showing the major structures within the organ of Corti. (Based on a drawing by Polyak, 1946.) From The Auditory System: Anatomy, Physiology, and Clinical Correlates (2nd edition, p. 101), by F. E. Musiek and J. A. Baran, 2020, San Diego, CA: Plural Publishing. Copyright © 2020 Plural Publishing, Inc. All rights reserved.
Figure 2–13. A micrograph from above the reticular lamina. (Courtesy of M. Pinheiro.) From The Auditory System: Anatomy, Physiology, and Clinical Correlates (2nd edition, p. 105), by F. E. Musiek and J. A. Baran, 2020, San Diego, CA: Plural Publishing. Copyright © 2020 Plural Publishing, Inc. All rights reserved.
The stereocilia of the hair cells protrude through the lamina. The reticular lamina is composed of phalanges of Deiter’s cells and inner and outer border cells, as well as the tops of the pillar cells. It keeps the endolymph from penetrating the internal structures of the organ of Corti. Hence, only the stereocilia and not the hair cells themselves are bathed in endolymph, which has a +80 mV charge (see Figure 2–13).
The lateral wall of the cochlear duct is discussed next. The lateral wall is the location of the stria vascularis and the spiral ligament (see Figure 2–9). The stria vascularis is thought to play key roles in the production and absorption of endolymph. It is composed of three layers of cells. Marginal cells make up the first layer and are linked to ion channels and pumps. The intermediate cell layer is lateral to the marginal cells and contains melanin, and the basal cell layer is next to the spiral ligament. The cells in all three cell layers are critical for the function of the cochlea. The stria vascularis also provides blood supply to the cochlea and maintains the metabolism of the inner ear (Slepecky, 1996).
The spiral ligament extends throughout the cochlea and covers the lateral wall of the scala media. It also extends inferiorly to the upper scala tympani. This structure supplies support to the lateral aspect of the BM as well as for Reissner’s membrane.
Sensory Cells. There two types of sensory cells in the cochlea: inner and outer hair cells (IHCs, OHCs). The OHCs are organized in multiple rows (3–5) and the IHCs have only one row. These hair cells are located on the lateral and medial sides of the pillar cells, respectively (Figure 2–14). There are about 3,500 IHCs and 12,000 OHCs that course the length of the cochlea. Stereocilia are located at the top of both types of hair cells. The OHC’s structure is different from that of the IHC (Figure 2–15). The OHCs are rather elongated tubelike structures that vary greatly in their length. The OHCs in the high-frequency regions of the cochlea are shorter than those located in the low-frequency regions (Geisler, 1998). Located within the OHCs are contractile proteins such as actin, myosin, prestin, and tubulin. There are also special structures along the outer walls of the OHCs called cisterns. These cisterns and chemicals allow for rapid expansion and contractile movements of the cells (discussed later). The OHCs usually have a −60 mV electrical charge and potassium ion channels. These cells have a cuticular plate on top that supports the stereocilia, which connect to the underside of the tectorial membrane. Each OHC has three rows of stereocilia with a greater number of stereocilia per cell at the base (approximately 150) than at the apex (approximately 50) of the cochlea. These stereocilia are stiff and form a W shape when looking down on the top of the hair cell.
The IHCs have a different structure than the OHCs (see Figure 2–15). They have more mitochondria and both calcium and potassium channels, and they do not have contractile proteins and cisterns. The IHCs have a −40 mV charge and about 50 to 70 stereocilia per cell, which are arranged in a U shape. The IHCs typically have three rows of stereocilia that are graded in length, and the stereocilia are longer at the apex of the organ of Corti than at its base.
Both the OHCs and IHCs have stereocilia that have pores that open when stimulated. This allows K+ ions to pass into the cell resulting in depolarization. Tip-links are small filament bands that open the pores on movement in a certain direction (see Figure 2–15). The tip-links are likely to be located toward the top of the stereocilia. Cross-links are similar in structure to tip-links, but they connect to the stereocilia closer to their midpoints (see Figure 2–15). This not only allows the stereocilia to move in unison, but it also supplies some support for this movement (Geisler, 1998). The hair cells are tuned so that cells located more basally respond to high-frequency sounds, whereas the more apically situated cells respond to lower frequency sounds. This arrangement of hair cells leads to the tonotopic representation of frequencies within the cochlea, with the higher frequency sounds being represented at the basal end of the cochlea and the lower frequency sounds at the apical end of the cochlea.
Figure 2–14. A photo showing the tunnel of Corti. Key: IHC = inner hair cell, OHC = outer hair cell. (Courtesy of R. Mount, Hospital for Sick Children, Toronto.) From The Auditory System: Anatomy, Physiology, and Clinical Correlates (2nd edition, p. 101), by F. E. Musiek and J. A. Baran, 2020, San Diego, CA: Plural Publishing. Copyright © 2020 Plural Publishing, Inc. All rights reserved.
Supporting Cells. Supporting cells are located along the organ of Corti and include pillar cells, Deiter’s cells, phalangeal cells, Hensen’s cells, Claudian cells, border cells, and Bottcher’s cells (Musiek & Baran, 2020; Slepecky, 1996) (see Figures 2–12 and 2–14). Next to the limbus are the border cells that divide the IHCs from the inner sulcus. The phalangeal cells support the IHCs and have stalks that separate these cells. These stalks or phalanges give rise to a flattened apical plate that contributes to the reticular lamina. A similar structure is observed in the case of the Deiter’s cells, which support the OHCs. The inner and outer pillar cells enclose the tunnel of Corti and, because of their triangular structure, they enhance the stiffness of this region where there is considerable movement of the BM. The space of Nuel is located next to the outer pillar cells. The lateral segment of the BM is where the Hensen’s cells, the cells of Claudius, and Bottcher’s cells are found. These all lend support to the lateral aspect of the BM. Between the most lateral OHCs and the Hensen’s cells is a space called the outer tunnel.
Figure 2–15. Sketches of the inner (A) and outer (B) hair cells. Key for (A): 1 = stereocilia, 2 = nucleus, 3 = mitochondria, 4 = tip-links, 5 = cross-links, 6 = afferent nerve terminal, 7 = efferent nerve terminal; key for (B): 1 = stereocilia, 2 = cisternae, 3 = mitochondria, 4 = cross-links, 5 = tip-links, 6 = pores, 7 = nucleus, 8 = efferent nerve terminal, 9 = afferent nerve terminal. From The Auditory System: Anatomy, Physiology, and Clinical Correlates (2nd edition, p. 108), by F. E. Musiek and J. A. Baran, 2020, San Diego, CA: Plural Publishing. Copyright © 2020 Plural Publishing, Inc. All rights reserved.
Function: The Membranous Cochlea
The physiology of the cochlea can be separated into two major areas of study: cochlear mechanics and cochlear electrophysiology. Although these two areas of function will be presented separately, it is important to realize that cochlear mechanics and electrophysiology are interdependent and function smoothly as part of a larger continuum that helps make up the hearing experience. Some key broad-based functions of the cochlea are (1) changing vibrotactile energy to electrical energy and (2) coding for intensity, frequency, and time. These functions require both mechanical and electrophysiologic processing.
Cochlear Mechanics. The ossicular chain’s vibratory energy is imparted to the cochlea through the stapes’ motion at the oval window. Key to starting the mechanical functions of the cochlea is that as the stapes pushes in (compression wave) or out (rarefaction wave) of the oval window, the round window membrane accommodates this movement. This interaction between the oval and round windows cannot be compromised without affecting the cochlear hearing processes.
The input from the stapes displaces the fluid in the cochlea in a manner that creates a traveling wave (TW) that moves down the length of the cochlea. This TW causes an activation of subsequent mechanical and electrochemical processes in the cochlea. It has been estimated that the TW moves nearly 100 msec at the most basal segment but slows down to about 2 msec at the apical most aspect of the cochlea (see Zwislocki, 2002). This velocity has implications for neural synchronization that are reflected in such measures as the auditory brainstem response (ABR). The ABR shows more synchronous activity derived from the basal end than the apical end of the BM.
Frequency can be coded in the cochlea in two ways, by place and by periodicity (temporally). The place theory, which was first described by von Békésy (1960), argued that sounds traveled down the BM and that frequency was coded by the point of maximum displacement of the BM by the traveling wave (TW), with high frequencies being represented more basally and low frequencies more apically (Figure 2–16). The TW reaches its maximum deflection at the place where the resonance of the BM matches that of the stimulating sound. This maximum deflection refers to the maximum amplitude point for the envelope of the TW (Figure 2–17). The difficulty with the place theory was that the tuning required for behavioral frequency discrimination was not consistent with the sharpness of the TW envelope; however, this problem was resolved with the discovery of the biological amplifier that will be discussed later.
Figure 2–16. A drawing showing the relationship between frequency and its location on the human basilar membrane. From The Auditory System: Anatomy, Physiology, and Clinical Correlates (2nd edition, p. 121), by F. E. Musiek and J. A. Baran, 2020, San Diego, CA: Plural Publishing. Copyright © 2020 Plural Publishing, Inc. All rights reserved.
Figure 2–17. A drawing of the location of the traveling wave on the basilar membrane for three frequencies. Note the envelopes (dotted lines) are not symmetric in their shapes. From The Auditory System: Anatomy, Physiology, and Clinical Correlates (2nd edition, p. 119), by F. E. Musiek and J. A. Baran, 2020, San Diego, CA: Plural Publishing. Copyright © 2020 Plural Publishing, Inc. All rights reserved.
Frequency also can be coded temporally. That is, the rapid movement of the BM (fine structure) is associated with the cycles of the acoustic stimulus that stimulate the hair cells and auditory nerve fibers accordingly. For example, if a 200-Hz tone were presented to the ear, the BM would move 200 times per sec and the hair cells and auditory nerve would be stimulated and fire at the same rate. This kind of frequency coding may work feasibly for low-frequency tones, but not well for high-frequency stimuli as the firing rates needed to code high-frequency sounds are likely to exceed the physiologic capacities of the hair cells. Hence, common thinking is that firing rate (i.e., temporal or periodicity processing) and place of maximum displacement work well for low frequencies but that, as the frequency of the stimulus increases, the place principle becomes more dominant (Geisler, 1998; Musiek & Baran, 2020; von Békésy, 1960; Yost, 2000).
Amplitude of the TW is how intensity is represented in the cochlea; that is, the greater the intensity of the signal, the greater the amplitude of the TW envelope. However, with increases in intensity, the displacement of the BM is not linear. The TW envelope has greater damping on the apical side; hence, the TW envelope has a sharper reduction in amplitude on the apical side than is reflected in the increase in amplitude on the basal side (see Figure 2–17). When intensity increases, not only does the vertical displacement increase, but so does the width of the envelope. This action results in more hair cells being stimulated, and in turn, more neural elements being activated. Because of the broader displacement of the envelope at high intensities, there is a decrease in the sharpness of the peak of the TW, resulting in poorer frequency selectivity.
Nonlinearity associated with the BM is an important concept. As mentioned earlier, as intensity increases, so does BM displacement, but not in a linear fashion. At low intensities, there is proportionately more displacement of the BM than at high intensities (Figure 2–18). Like intensity, the representation of frequency on the BM is not linear. An octave change for a high-frequency sound on the basal part of the BM requires less space than an octave change at the low-frequency end of the BM. This nonlinearity can be viewed as a natural “compression” within the cochlea for both intensity and frequency (see Musiek & Baran, 2020; Yost, 2000).
Hair Cell Mechanics. One of the hair cells’ main functions is to convert vibratory energy into electrical energy so that the nervous system can utilize these signals. This mechanical activity starts at the stereocilia. The OHC’s stereocilia are embedded in the underside of the tectorial membrane. If a TW is a compression wave, the BM will be deflected downward. Because the tectorial membrane and the BM are attached at different sites, a downward movement of the BM will result in the tectorial membrane pulling the stereocilia toward the limbus (or medial aspect of the organ of Corti). This movement at the stereocilia level results in the tip-links closing the pores of the stereocilia. As a result, no chemical transduction can evolve and the hair cell cannot depolarize. However, when the BM moves upward, such as is seen when the stimulus is a rarefaction wave, the tectorial membrane pulls the stereocilia in the opposite direction and the pores open up, allowing K+ ions to enter the cell, starting the depolarizing process (Figure 2–19). The stereocilia essentially move in unison because of the cross-link connections among the stereocilia (Geisler, 1998).
Figure 2–18. A drawing showing basilar membrane displacements related to intensity increments of the stimulus. From The Auditory System: Anatomy, Physiology, and Clinical Correlates (2nd edition, p. 124), by F. E. Musiek and J. A. Baran, 2020, San Diego, CA: Plural Publishing. Copyright © 2020 Plural Publishing, Inc. All rights reserved.
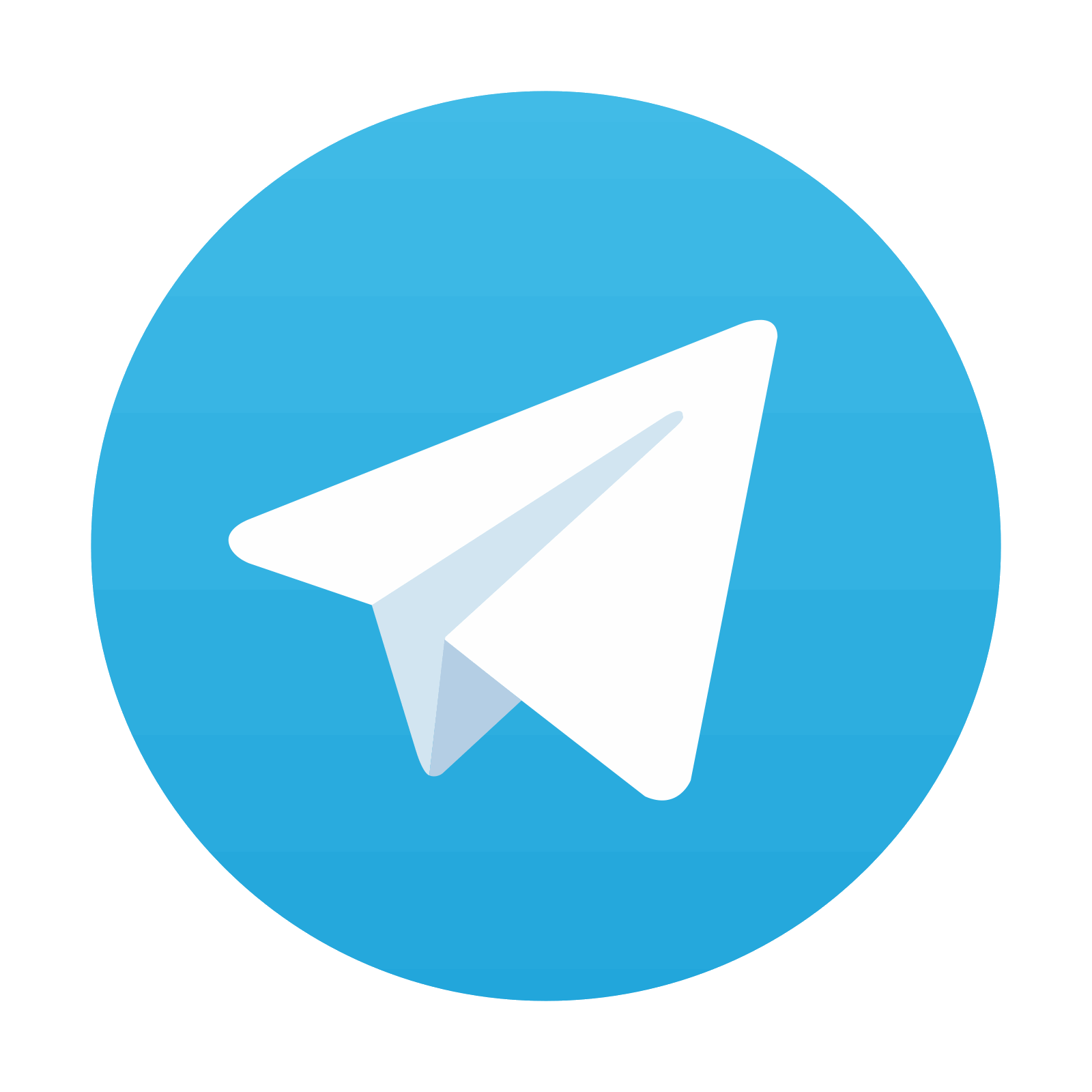
Stay updated, free articles. Join our Telegram channel
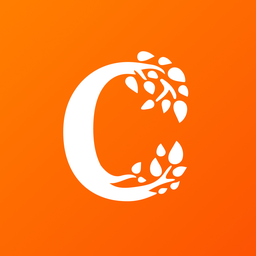
Full access? Get Clinical Tree
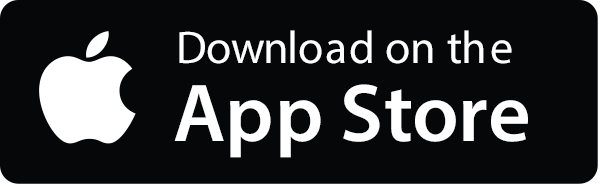
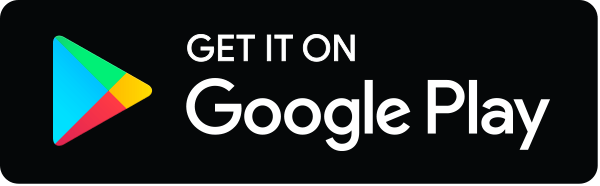