25 Stereotactic Radiosurgery and Fractionated Radiation for Pituitary Tumors
The use of radiation for scientific and medical purposes dates back over 100 years with the discovery of the x-ray by Conrad Roentgen in 18951 and the isolation of radium by Madame and Pierre Curie in 1898.2 Early external-beam techniques were developed for clinical use, but these devices produced very low-energy x-rays resulting in poor tissue penetration and severe skin damage. In the 1920s, Coutard and Regaud showed that by dividing the total dose into many smaller treatments, delivered over several weeks, tumors could be cured without severe normal tissue damage.3 This method was quickly adopted by others and ushered in as the concept of dose fractionation. The start of the modern era of external-beam radiation therapy began with the appearance of the cobalt-60 machines in the mid-1950s. Cobalt machines were less complex and delivered high-energy rays in the megavoltage (MV) range. Throughout the 1960s and 1970s, cobalt machines gave way to linear accelerators. In addition to photons, linear accelerators are able to produce electrons, which have a finite range in tissue that allows tailoring the depth of penetration to the exact clinical situation. Other advantages of linear accelerators include increased precision and variable dose rates. The technology of radiation delivery continues to undergo rapid development and change.
Physical Properties of Radiation
Ionizing radiation is composed of particulate radiation and electromagnetic radiation. Although some radiation treatments use electrons, protons, or neutrons (particulate radiation), the vast majority of radiation treatments are delivered via photons (electromagnetic radiation). Electromagnetic radiation is part of a continuum of waveform energy known as the electromagnetic spectrum. Near the high-energy (short wavelength) end of this spectrum lie the electromagnetic waves used therapeutically (x-rays, gamma rays) to treat patients. Gamma rays and x-rays are essentially identical, with the only difference being their origin. X-rays are obtained from an extranuclear process when an accelerated electron strikes a target with a high atomic number, causing deceleration of the electron with the production of polyenergetic x-rays through the process of “bremsstrahlung” (braking radiation). In contrast, gamma rays are emitted from the nucleus during nuclear decay.
High-energy electromagnetic radiation interacts with matter, removing electrons from their orbits, resulting in ionization and the release of energy. In this process the photon interacts with an outer shell electron, resulting in the release of the electron and a photon of lower energy deflected from its original course (Compton effect). These interactions are not dependent on the atomic number of the substance with which the x-ray is interacting, but only on the density of the absorbing material. The amount of radiation absorbed is roughly equal, gram for gram, whether the material is soft tissue or bone. This explains why body lead is protective in the energies typically used for diagnostic radiology but is ineffective with the higher energies typically used in radio-surgery and radiation therapy.
Electrons are the most commonly used particulate radiation in general radiotherapy practice. However, due to the lack of penetration of electrons, they are rarely used in the treatment of intracranial diseases. In contrast, other forms of particulate radiation, such as protons and neutrons, are utilized in the treatment of intracranial diseases. Neutrons interact with the nuclei of atoms and produce much heavier particles, such as protons, α-particles (nuclei of helium atoms), and even heavier nuclear fragments. The density of ionizations along the pathway of these particles is much greater than that of x-rays. In contrast to neutrons, the advantage of protons is in their dose distribution.4 Protons deposit most of their energy at the end of their track range in tissue, called the Bragg peak. The energy delivered to tissue proximal to the peak is minimal. The energy delivered at the peak is high, and essentially none passes beyond the peak. This allows energy to be deposited in large amounts in a region while sparing the adjacent tissues. However, due to the complexity of particle beam treatment planning, the need for a cyclotron to generate the protons, and the expense of these units, there are only a limited number of facilities around the world.
Biologic Properties of Radiation
When photon radiation hits a cell it will most likely interact with water because cells are primarily composed of water. This creates unstable molecules called free radicals that may interact with DNA. Free radicals damage DNA and can result in permanent cell injury or death. This is called the indirect effect of radiation. Less commonly, photon radiation may interact directly with DNA, or other cellular molecules, leading to damage and possibly cell death. This direct effect is the predominant process in heavy particle radiation.
Cell Death from Radiation
Radiation of the cell leads to damage of the DNA, but not all of the damage is equally capable of producing biologic injury. DNA—protein cross-links, direct base damage, and single-strand breaks seem to play a relatively minor role in the lethality of ionizing radiation compared with double-strand breaks.5 Provided the opposite strand is intact, cells remain capable of repairing single-strand breaks with great efficiency. Therefore, the critical lesions caused by radiation are double-strand breaks. The most common types of cell death after radiotherapy are mitotic cell death and apoptosis. If a cell accumulates sufficient radiation damage, its later progeny may be unable to go through mitosis successfully and will die. Several cell divisions may occur before the lethal effects of radiation are expressed. Consequently, rapidly dividing normal tissues (i.e., oral mucosa) or malignant tumors express radiation damage sooner than slower dividing normal tissues (i.e., brain) or benign tumors such as pituitary adenomas. Some cells do not have to go through mitosis to succumb to radiation but instead die from programmed cell death or apoptosis.6 An apoptotic event is irreversible, and there is no capability for repair of radiation damage, which characterizes cells dying from mitotically expressed DNA damage.
Therapeutic Ratio
To have a therapeutic effect, radiation must differentially damage a tumor more than the adjacent normal tissues. This is accomplished physically by designing radiation treatments that deliver high doses of radiation to the tumor with less radiation going to nearby structures. The therapeutic ratio can also be improved biologically by utilizing the advantages of differential radiosensitivity of normal tissues and tumors with dose fractionation. In general, normal tissues are better able to repair DNA damage than tumors because of the aberrant cell cycle control mechanisms in tumors. However, at the doses typically used for radiosurgery the repair mechanisms of even normal tissues can be overwhelmed, and radiation damage can occur along with the desired effect on the tumor.7 Therefore, unless the radiation dose delivery is highly conformal, it is advantageous to deliver multiple small doses of radiation because of the normal cells’ inherent superiority in repairing sublethal damage compared with tumor cells.
Fractionated Radiation Therapy
Proper immobilization and consistent reproducibility of the treatment position are essential for patients undergoing radiotherapy. Therefore, a device is needed to accurately reposition the patient on a day-to-day basis. Most radiation therapy centers fabricate a thermoplastic mask for each patient to prevent the head from moving during treatment. To provide consistent references points, fiducial markers are placed on the mask. Each day during treatment setup, these markers are aligned with lasers in the linear accelerator treatment room, allowing precise alignment of patients on a daily basis. Because the treatment may last several weeks, a repeatable head fixation device is an absolute requirement for any facility performing stereotactic radiation therapy (SRT). These devices often include a relocatable head frame stabilized by a mouth-guard–type bite block. These “pinless” stereotactic systems can achieve an accuracy of better than 2 mm.
Once an immobilization device has been constructed, the patient undergoes a treatment simulation and planning session in the treatment position. Historically, this was performed using conventional fluoroscopic units to visualize bony anatomical landmarks. More recently, simulators using computed tomography (CT) to image the region of interest are obtained to plan the radiation treatments. The planning CT scanners are similar to diagnostic scanners, except that planning scans are conducted under the same conditions (on a flat table with custom immobilization devices) used for treatment of the patient on the linear accelerator. The CT simulators incorporate workstations allowing efficient target contouring, image manipulation, and simulation of beam arrangements. Image fusion between CT and other imaging modalities, such as magnetic resonance imaging (MRI), can also be performed on these workstations.
Once the planning CT images are in the treatment planning computer, the radiation oncologist defines the gross tumor volume, volumes at risk for tumor spread (clinical target volume [CTV]) without obvious gross disease on imaging, and critical normal structures. A planning target volume (PTV) is then generated by enlarging the CTV with a margin of approximately 2 to 4 mm to account for patient setup error and inaccuracies in imaging. A treatment plan is then a constructed plan to deliver a homogeneous radiation dose to cover the PTV while minimizing the dose to critical structures outside the PTV. By achieving a homogeneous dose distribution, areas of excessive dose are avoided, thereby decreasing the risk of radiation damage. The radiation oncologist reviews the computerized dose distributions (isodose lines) displayed and determines whether the plan is acceptable or in need of modification.
With the advent of sophisticated computerized planning systems and advances in neuroimaging, it is now possible to deliver high does of radiation to the sellar region while sparing the surrounding normal structures from these high doses of radiation. Methods to accomplish this dosimetric goal include the use of multiple, nonoverlapping, conformal radiation beams shaped by custom-made blocks to the configuration of the tumor volume, also called three-dimensional (3D) conformal radiotherapy. Computer-controlled tungsten leaves, or multileaf collimators, are individual leaves that shift to form specific patterns, conforming the radiation beam to the target. In addition to blocks, wedges are often added to alter the radiation beam dose distribution to allow more uniform dose distribution within the treatment volume.
Another recent innovation, intensity modulated radiation therapy (IMRT), provides a completely different approach to treatment planning. Instead of clinicians defining beam directions, beam weights, wedges, blocks, and margins, and then computing and displaying dose distributions to assess whether the treatment plan will lead to an acceptable outcome, they do the opposite or “inverse” treatment planning. The radiation oncologist specifies the prescribed target dose and the dose constraints for normal structures. Sophisticated algorithms of the inverse treatment planning system enable the evaluation of millions of possible beam arrangements and determine the clinically optimized treatment plan, maximizing the dose to the tumor while minimizing exposure to surrounding healthy tissue. Hundreds to thousands of small, modulated radiation beams strike a tumor site with varying intensities, and from many angles, to attack the target in a complete 3D manner. A comparison of linear accelerator–based radiosurgery, fixed noncoplanar 3D custom blocked fields, and IMRT did find improved dose conformity and decreased dose to nontarget brain in high and low isodose regions for the IMRT plans for the irregular tumor targets.8
Typically, the patient receives five treatments a week, one a day, Monday through Friday. Along with these daily treatments, most centers utilizing stereotactic radiotherapy also utilize image-guided radiation therapy (IGRT), which involves two-dimensional (2D) or 3D imaging that is obtained before treatment to direct the radiation therapy to the correct location with high precision. An example of 3D IGRT is cone-beam computed tomography, which acquires many 2D projections over the entire volume of interest in each projection. These projections are then reconstructed into a 3D volume analogous to the CT planning data set and then used to align the patient into the correct position.
An example of 2D IGRT is obtaining an orthogonal pair of kilovoltage radiographs before treatment, then using an automated matching system, the patient is moved robotically to correct patient setup errors and to track any patient movement throughout the treatment.
Most conventional fractionation schedules are delivered with 1.8 to 2 Gy fractions daily. Smaller doses per fraction allow more healing of sublethal damage between treatments. Hypofractionation, using fewer fractions but larger doses per fraction (3 to 7 Gy) to achieve a lower total dose (20 to 30 Gy), has also been employed by some centers. Despite the theoretical radiobiologic equivalence of such hypofractionated schedules with either conventional dose fractionation or high-dose, single-fraction techniques, there are no long-term studies on tumor control or toxicities.
Stereotactic Radiosurgery
Stereotactic radiosurgery is a surgical procedure that combines the principles of stereotactic localization with the precise delivery of radiation to an imaging-defined target. Lars Leksell,9 neurosurgeon at the Karolinska Institute in Stockholm, Sweden, conceived the concept of stereotactic radio-surgery in 1951. His goal was to develop a method for the noninvasive destruction of intracranial lesions that were at high risk for surgical resection. To accomplish this goal, Leksell10 coupled stereotactic localization with the delivery of a large, single-fraction dose of ionizing radiation. In 1968 Leksell and Börje Larsson developed the first cobalt-60 gamma unit (the Gamma Knife, Elekta Instruments, Norcross, GA). Over the past two decades radiosurgery has gained acceptance and has become an integral part of both neurosurgery and radiation oncology. Advances in neuroimaging and improved computer software have made radiosurgery safer and more effective in the management of a wide range of disorders affecting the nervous system. Today, radiosurgery is available to patients at the majority of large medical centers.
The goal of radiosurgery is to deliver a high radiation dose to an imaging-defined target. In contrast to conventional fractionated radiation therapy, radiosurgery does not rely on the increased radiation sensitivity of the target compared with the normal brain. Instead, radiosurgery spares normal structures using conformal dose plans that deposit large radiation doses into the target with a rapid fall-off of radiation at the edges of the dose plan (Fig. 25.1). Different radiosurgical systems satisfy these essential criteria by using various technical solutions.
Leksell Gamma Knife
Several versions of the Leksell gamma knife have been developed over time with the goals of increased precision and accuracy of radiation delivery. Recently the Perfexion (Elekta Instruments, Norcross, GA) was introduced as the latest version of this device. It contains 192 individual cobalt- 60 sources that naturally decay to nickel (60
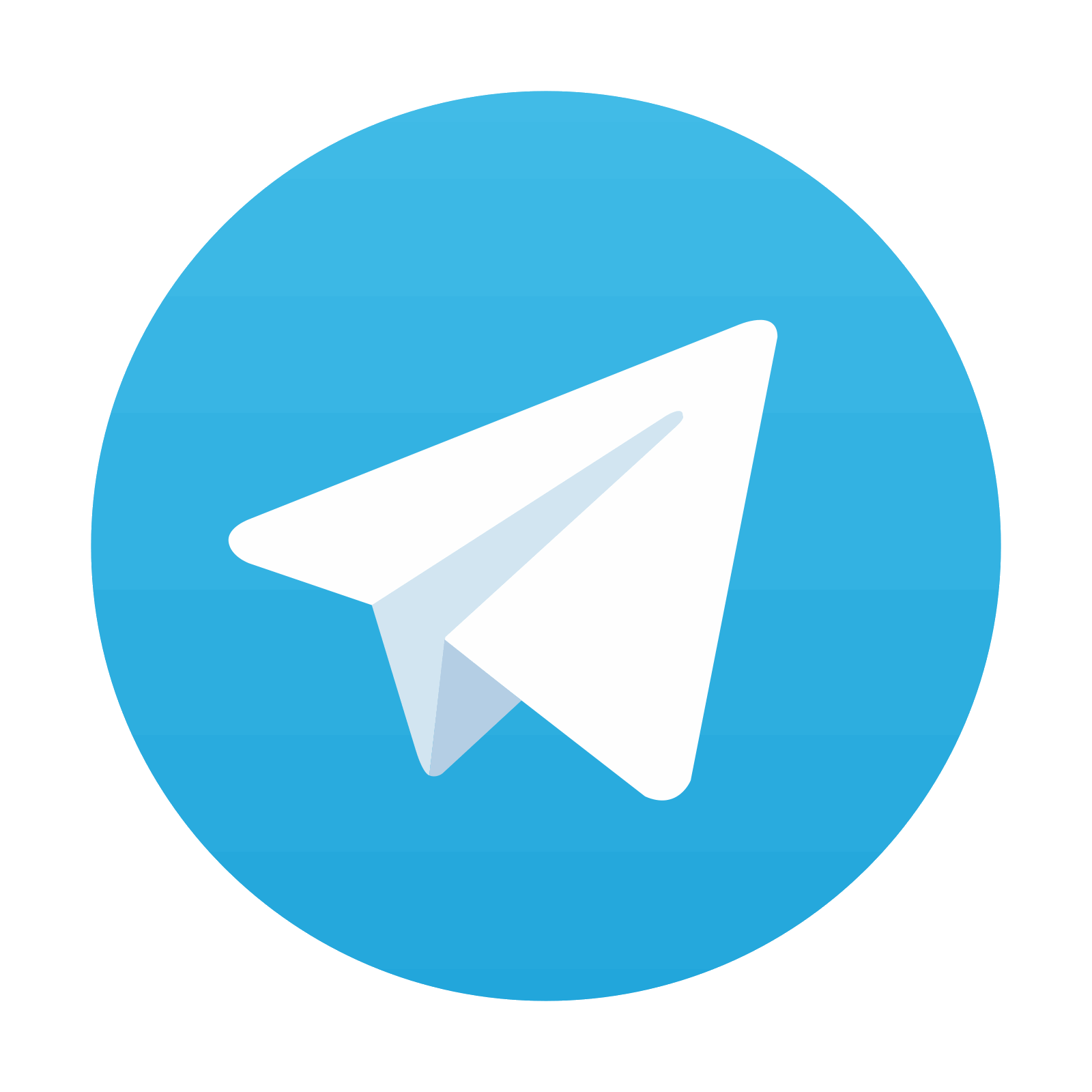
Stay updated, free articles. Join our Telegram channel
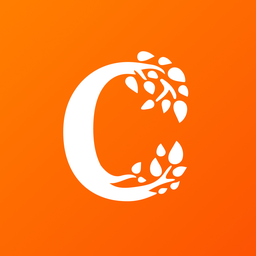
Full access? Get Clinical Tree
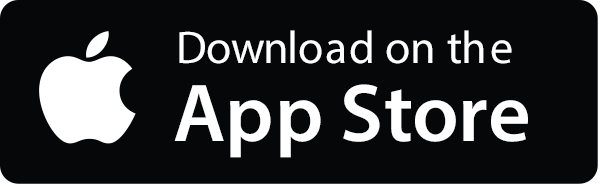
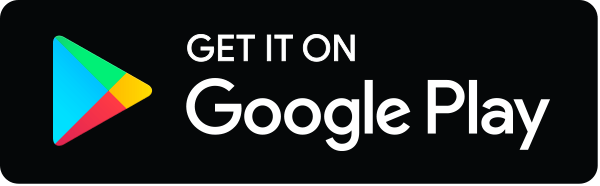