Overview
Retinal photoreceptors are paradoxically efficient in their ability to capture photons for visual transduction while being vulnerable to cellular damage from excess light. Although the molecular mechanism of visual transduction is now relatively well understood, our understanding of retinal phototoxicity, or retinal light damage, is less complete. Nonetheless, based to a degree on comparable end-stage morphology, retinal photic injury in laboratory animals has served as a model of retinal degenerations of genetic origin, aging, and from light-induced trauma during ocular surgery for over 40 years. Likewise, a long-standing clinical interest in the potential for interactions between diet, light environment, and retinal injury has prompted studies with animal models. But are animal models of light-induced retinal degeneration appropriate models for human retinal disease? Furthermore, although high levels of light accelerate some forms of inherited retinal degeneration and might influence the incidence of age-related macular degeneration (AMD), what role does light environment play in retinal disease?
Clinical background
Light duration and intensity
Visible light-mediated photoreceptor cell damage is a photochemical process with a well-known inverse time and intensity relationship. This effect, often referred to as reciprocity, implies that a longer-duration light exposure can be shortened by the use of higher-intensity light, and vice versa. This concept is well recognized in anterior-segment surgery and during vitrectomy where light intensity and time-dependent phototoxic lesions have been reported. These lesions appear hours to days after surgery and are often seen in or near the cone-rich foveal region of the eye. The outcome for most phototoxic lesions found outside the fovea is generally good while the prognosis for repair of foveal damage, more often seen following vitrectomy, is less favorable. Solar retinitis also presents with foveal damage. It is well known to occur in patients deliberately sungazing for extended periods, but a few cases have been reported following relatively short periods of sunbathing in high (often reflective) light environments. Likewise, photic injury from an indirect ophthalmoscope following relatively brief exposure has been reported. There are limits on photochemical damage in the retina and reciprocity, however, in that short-duration high-intensity coherent light actually results in thermal damage. Discussion of laser light-induced damage, sometimes employed in treating choroidal neovascularization and routinely used in the treatment of retinal breaks, is beyond the scope of this chapter.
Spectral characteristics of damaging light
Retinal light damage is also wavelength-dependent, with green light most effectively causing damage in rod photoreceptors. In other words, the action spectrum for light damage closely approximates the absorption spectrum for rhodopsin. ( Figure 64.1 ). However, blue light as well as full-spectrum white light also causes retinal damage by bleaching rhodopsin, albeit less efficiently. Blue light has a shorter wavelength than green light and is therefore more energetic (Planck’s relation: E = h/λ, where E = energy, h = the Planck constant, and λ = wavelength). Accordingly, blue light is capable of photoisomerizing free all -trans retinal, the aldehyde form of vitamin A, into the 11- cis stereoisomer required for rhodopsin regeneration. This reaction may short circuit the normal postrhodopsin-bleaching vitamin A cycle, involving migration of all -trans retinol into the retinal pigment epithelium (RPE) and enzymatic reisomerization to the 11- cis form. In primates, cone cells undergo damage from short intermittent pulses of blue light, which has also been attributed to rapid bleaching and regeneration of blue-cone visual pigment. RPE cell damage from blue light exposure probably involves different chromophores. For example, mitochondrial cytochrome proteins have been suggested as one chromophore, and a potential source for the generation of reactive oxygen species. Another potential source of blue light-mediated reactive oxygen in RPE is photosensitization of bis-retinaldehyde-phosphatidylethanolamine (A2E), a lipophilic molecule that accumulates with age. Epidemiological studies indicate that chronic light exposure may be a risk factor for the development of AMD, but this effect seems to be small compared to the risk factors of age (>70 years), race (caucasian), and smoking. Light exposure might indirectly predispose to AMD through a variety of mechanisms (see Chapter 68 ). For example, Zhou and coworkers have suggested that products of the photo-oxidation of bis -retinoid lipofuscin pigments in RPE cells may serve as a trigger for the complement system ( Box 64.1 ).

- •
Light damage is a photochemical process: exposure duration and light intensity inversely affect the degree of damage (reciprocity)
- •
Action spectrum for light damage closely approximates the absorption spectrum for rhodopsin
- •
Green light causes damage most efficiently in rod photoreceptors
- •
Blue light can cause retinal damage by inducing photoisomerization of free retinaldehyde, which promotes more rapid rate of rhodopsin regeneration and subsequent rebleaching
- •
Blue light-mediated retinal pigment epithelium damage may involve mitochondrial cytochrome proteins and/or photosensitization via bis -retinaldehyde phosphatidylethanolamine (A2E)
Pathology
Photoreceptor cells are differentiated postmitotic retinal neurons that normally function throughout the life of an individual. Some visual cell dropout occurs naturally as a result of aging, but rod and cone survival into the seventh decade and beyond is normally more than sufficient to preserve vision. A higher rate of visual cell loss is seen in many genetic conditions, but the rate of loss is still relatively slow compared to most experimental animal models of retinal degeneration. At the same time the morphological endpoints of both genetic and experimental retinal degenerations are so similar that they invite comparisons and inferences into etiology and prevention based primarily on studies with animals. In the case of photic injury in animal models, one sees loss of rod photoreceptors, with a relative sparing of cones. Rod cell loss is graded, most pronounced in the central superior hemisphere, and often particularly severe in the region 1–2 mm superior to the optic nerve head. At least initially, Müller cells and the inner retinal neurons, consisting of horizontal, bipolar, amacrine, and ganglion cells, appear to be immune to light. The reasons for this dichotomy are unknown; however, it is not a simple lack of cellular chromophores, as light-absorbing proteins such as melanopsin and cryptochromes are present in the inner retinal layers. Recently, it was suggested that the tripeptide glutathione may be absent, or nearly so, from photoreceptor cells while present in sufficient quantities in the inner retinal layers to prevent oxidative damage. As in some animal models, rod cell degeneration precedes that of cone cells in the early stages of AMD. However, in AMD the loss of both rods and cones appears to result from RPE dysfunction in the parafoveal/macular region. Irrespective of the reasons for their susceptibility, when photoreceptor cell loss is extensive remarkable changes in inner retinal neuron morphology, location, and synaptic function soon follow ( Box 64.2 ).
- •
Loss of rod photoreceptors with relative sparing of cones
- •
Inner retina (bipolar, horizontal, amacrine, ganglion cells) initially spared
- •
Extensive photoreceptor cell loss leads to changes in inner retina neuron morphology, location, and synaptic function
Etiology
Genetic risk factors
Under ordinary conditions, eye pigmentation and pupil size play major roles in preventing photic injury. By constricting pupil diameter during high light (photopic) conditions much of the light entering the eye is focused on the cone-rich fovea, which is relatively resistant to damage. Because melanin in the RPE absorbs light that passes entirely through the retina, its presence may help to reduce light scatter and photosensitized reactions in the choriocapillaris or in Bruch’s membrane. In addition, because the RPE cell layer covers the entire globe of the eye and melanin pigments are present in the iris, ciliary body, and choroid, extraneous light is largely prevented from entering the eye through the sclera. In fact, melanin is such an effective filter that, for photic damage to occur in pigmented animals, mydriatics are usually required. In low light (scotopic) conditions the pupils are also dilated, allowing a larger area of the retina to be illuminated, but the intensity of light is much reduced, as is the chance of retinal injury. Pigmented animals have the same action spectrum for retinal light damage ( Figure 64.1 ) and exhibit the same morphological characteristics as nonpigmented animals. However, because albino strains lack melanin, they are approximately twofold more sensitive to photic injury.
Other genetic mutations also exacerbate the effects of light exposure on the rate of retinal degeneration. For example, the most common form of autosomal-dominant retinitis pigmentosa (RP) involves a histidine for proline substitution at amino acid position 23 in rhodopsin (P23H). In patients having the P23H mutation, external factors appear to affect the rate of functional loss of both scotopic and photopic vision. Heckenlively et al found that patients working in bright light environments lost vision sooner than others working in dim light environments. In transgenic animal models with the P23H mutation, rod cell loss also precedes that of cone cells, and the rate of loss also depends on lighting conditions. While the P23H animal model does not mimic all of the electrophysiological findings in RP, it correctly predicts the effects of light environment on the rate of retinal degeneration and has provided insights into the mislocalization of mutated rhodopsin in rod outer-segment disks. Similarly, Royal College of Surgeons (RCS) rats exhibit a retinal degeneration that depends on environmental light. Dark rearing delays the onset of photoreceptor cell loss, while normal light exposure greatly accelerates that loss. As they age, RCS rats also accumulate a partially degraded cellular “debris” layer between the RPE and retina, which results from an inability of RPE to phagocytose rod outer-segment material. This debris layer forms a barrier that impedes the exchange of metabolites and the flow of nutrients from choroid to retina, leading to cell death. Morphologically, the end-stage RCS retina resembles that of several human retinal diseases, but whether the inhibition of nutrient flow is an appropriate model for human disease is currently unknown. A number of mouse strains also present with variable light damage sensitivities. In several strains, their susceptibility to retinal photic damage has been shown to correlate with a mutation in RPE 65, an enzyme involved in vitamin A (retinol) processing. Mice having the RPE 65 Leu 450 variant are more easily damaged, while mice with the RPE 65 Met 450 variant are more resistant to the phototoxic effects of light ( Box 64.3 ).
- •
Melanin acts as screening pigment to reduce light effects on the retina
- •
Genetic animal models mimic some aspects of human retinal disease
- •
Vitamin A metabolism and dietary fatty acids affect retinal development and susceptibility to light damage
- •
The polyunsaturated fatty acid docosahexaenoic acid can be converted into two different oxidative products
Dietary factors
Animal models lend themselves particularly well to understanding the role of diet on retinal development and disease progression. The effects of dietary retinol on rhodopsin levels and retinal light damage have been described for vitamin A-deficient rats and after inhibition of the visual cycle with 13- cis retinoic acid. In each case, a reduced level of available 11- cis -retinaldehyde leads to an increase in opsin levels and to reduced light damage susceptibility. A similar decrease in rhodopsin levels and increase in opsin in patients treated for acne with 13- cis retinoids is thought to lead to the development of deficits in scotopic vision. Insufficient dietary linolenic acid (18:3n-3), the essential fatty acid precursor of docosahexaenoic acid (22:6n-3; DHA), leads to a decrease in retinal DHA and to a decrease in susceptibility to photic damage. The amount of DHA in the retina is species-specific, but in rat rod outer segments it may represent as much as 50 mol% of the total. In other words, one of every two fatty acids found in rod outer-segment lipids can be a polyunsaturated DHA. Rod outer segments from transgenic P23H rats contain lower levels of DHA than normal, but unpredictably these animals are more sensitive to the damaging effects of light than normal rats. Furthermore, feeding P23H rats diets high in omega-3 fatty acids has not proven to be effective in delaying photoreceptor cell loss. Some forms of RP are associated with low DHA levels in red blood cells, but in these cases dietary DHA seems to be beneficial. Thus, a link between the reduced levels of DHA in rod outer segments of transgenic animal models and the low circulating levels of DHA in some RP patients has not yet been established.
The importance of dietary omega-3 fatty acids during visual system development, however, has been established, for rats and for primates, and, more recently, for preterm infants. Following clinical trials with full-term infants, DHA is now routinely added to infant formulas, along with the omega-6 fatty acid, arachidonic acid. Dietary supplementation with these fatty acids has a positive outcome on the development of vision and, although total dietary fat intake is associated with AMD, omega-3 fatty acids appear to slow disease progression. Accordingly, as a part of the ongoing Age-Related Eye Disease Study (AREDS) II, DHA supplementation is now being tested in patients. A rationale for this clinical trial appears to be based on the neuroprotective effects of this omega-3 polyunsaturated fatty acid. In the RPE, small amounts of DHA are enzymatically converted to neuroprotectin D1 (NPD-1), which has been shown to be effective in reducing apoptotic cell death. However, DHA is also capable of being oxidized by molecular oxygen, leading to the formation of carboxyethylpyrrole (CEP) protein adducts. NPD-1 is a 22-carbon DHA derivative containing two hydroxyl groups, while CEP is a 7-carbon DHA oxidation fragment, with a pyrrole ring adducted to the amine side chain of amino acids such as lysine ( Figure 64.2 ). Crabb and associates analyzed drusen dissected from the RPE layer of AMD and age-matched controls and found higher than normal levels of these covalent adducts in AMD eyes. Recently a mouse model of AMD, prepared by immunization with CEP adducted to albumin, has been shown to exhibit RPE deposits and pathology. This model and others may be useful in preclinical studies to help sort out the protective and damaging effects of dietary DHA and its derivatives.

Pathophysiology
Initiation of retinal light damage
Mechanistically, the principal utility of the photic injury animal model resides in the synchronous involvement of rod photoreceptors. Unlike genetic or age-related conditions, where small numbers of visual cells are typically involved at any one time, nearly the entire complement of photoreceptors is affected by intense light. Noell et al were the first to report that extensive rhodopsin bleaching is the trigger for retinal light damage. Genetic modification of photoreceptor cell proteins also points to rhodopsin as the key mediator of retinal damage by light. Rhodopsin knockout (KO) and RPE-65 KO mice are both protected against photic injury. Because opsin, but not rhodopsin, is present in RPE-65 mice and rhodopsin KO mice lack the protein altogether, the rate of rhodopsin regeneration during light has been implicated in the damage mechanism. Ironically, whole-body hyperthermia during light exposure also accelerates rhodopsin regeneration and greatly exacerbates retinal light damage. Photic injury occurs under ordinary room light in arrestin KO mice and rhodopsin kinase KO mice, while dark rearing prevents damage. Arrestin and rhodopsin kinase both interact with light-activated rhodopsin and are involved in attenuating the photo response, implicating two additional visual transduction proteins in the damage mechanism. Young RCS rats are genetically predisposed to retinal light damage, which correlates with the relative expression levels of arrestin and transducin. In both RCS and normal rats, dark rearing leads to increases in transducin mRNA and protein levels and enhances light damage, while a normal cyclic light environment increases arrestin levels and reduces susceptibility to damage. Other genetic evidence suggests that two distinct pathways of retinal damage may exist, one involving transducin and relatively low light exposure levels and a second pathway involving high light levels and the transcription factor activator protein-1.
Antioxidants and oxidation
Compelling evidence exists that oxidative stress is an integral part of the light damage process, as both natural and synthetic antioxidants prevent photoreceptor cell damage and loss. Oxidation also appears to be a relatively early event in the damage process. Light-induced oxidation occurs within minutes in isolated photoreceptor cells, and the rapid appearance of blue light-induced reactive oxygen species, originating from mitochondria, has been reported. Lipid hydroperoxides in retina were found to be elevated in normal rats exposed to green light, as well as in an oxidatively susceptible, drug-induced rat model of Smith–Lemli–Opitz syndrome. The synthetic antioxidant dimethylthiourea (DMTU) was effective in preventing light damage when administered before lights on, but is ineffective when given after the onset of light ( Figure 64.3 ). Retinal protein markers of oxidative stress are also altered by light and impacted by antioxidants. The expression of retinal heme oxygenase (HO-1) increases after intense light exposure. HO-1 is a 32-kDa inducible stress protein and the first enzyme in a pathway involved in converting a pro-oxidant heme into bilirubin, an antioxidant. Retinol dehydrogenase (RDH), an oxidatively sensitive rod outer-segment enzyme that converts retinaldehyde into retinol, is partially inhibited by light exposure. However, pretreatment of rats with DMTU prevents both the light-driven increase in HO-1 expression and the decrease in RDH activity. In mice, inhibition of nitric oxide formation has been shown to reduce retinal light damage, further implicating oxidative stress in retinal phototoxicity ( Box 64.4 ).

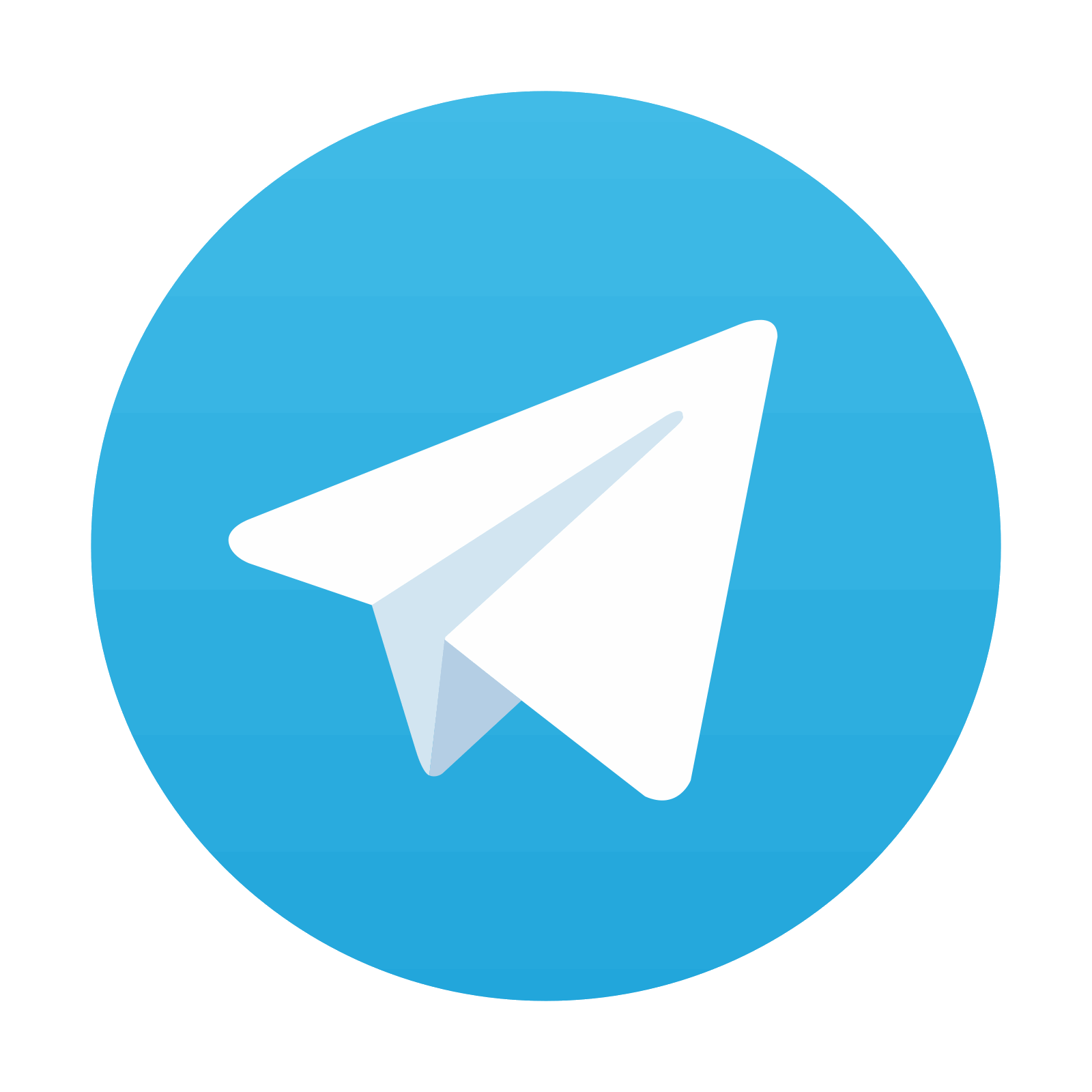
Stay updated, free articles. Join our Telegram channel
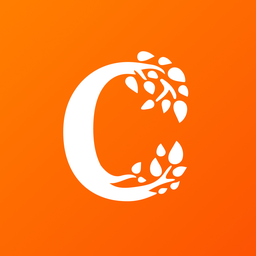
Full access? Get Clinical Tree
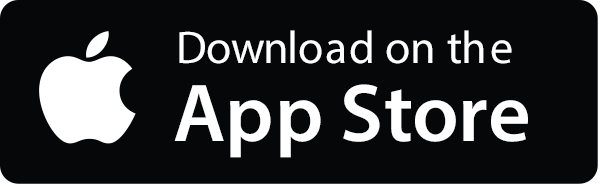
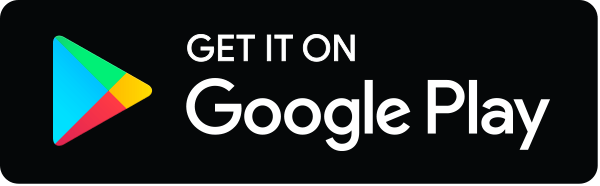
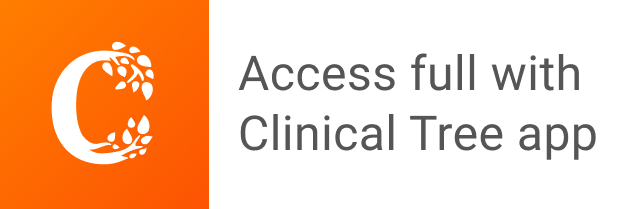