Clinical background ( Box 73.1 )
Altered retinal oxygenation plays a central role in the pathogenesis of many retinal diseases and a hypothesized role in others. Unfortunately, despite decades of work in some cases, the precise role of oxygen in certain complex diseases remains elusive.
Retinal diseases are complex, but hypoxia contributes to many:
- •
Diabetic retinopathy: hypoxia due to capillary loss in preproliferative retinopathy contributes to neovascularization. Possible hypoxia in background retinopathy
- •
Retinopathy of prematurity: hypoxia following end of oxygen supplementation contributes to neovascularization
- •
Central or branch retinal artery occlusion: choroidal supply cannot compensate for the lack of retinal circulation, leading to cell death in the inner retina
- •
Retinal detachment: separation of retina from choroidal circulation reduces flux of oxygen to photoreceptors
- •
Age-related macular degeneration: reduced choroidal blood flow and impaired transport may contribute to upregulation of vascular endothelial growth factor
- •
Glaucoma: reduced blood flow to the optic nerve head may contribute to hypoxia and the loss of retinal ganglion cells
There are three different categories of situations in which oxygen can play a critical role in disease. First, and most obvious, are situations involving ischemia. Because oxygen is usually the limiting substrate for metabolism, and cannot be stored, even a short-term loss of oxygen leads to devastating consequences in conditions such as central or branch retinal artery occlusion ( Chapter 63 ). Ischemia is certainly involved in other cases, such as after capillary dropout in diabetes ( Chapter 65 , Chapter 66 ). These conditions involve anoxia, because there is essentially no redundancy or safety factor in the retinal circulation where one arteriole or capillary can take over oxygenation of a region ordinarily supplied by another vessel. The choroidal circulation is usually not affected in the same diseases, but cannot compensate for the loss of retinal circulation when an individual is breathing air.
The extent to which substrates other than oxygen are involved in ischemic diseases is not generally clear. During retinal artery or capillary occlusion, one might expect that glucose from the choroid could diffuse into the inner retina to allow glycolytic metabolism, but this is uncertain. Purely glycolytic metabolism also leads to acidosis, which is observed in animal models of retinal artery occlusion. Interestingly, in primates and cats, retinal function does return to a large extent if circulation is restored within about 1.5 hours, which is far longer than the brain could survive. The long survival time probably does not reflect an inherent difference in retinal and brain neurons, but rather the small reservoir of glucose in the vitreous and the remaining supply from the choroid, neither of which has an analog in the brain. Elevating glucose prior to ischemia in rats prolongs the survival of the electroretinogram during ischemia, providing some support for this idea.
Some investigators believe that less severe reductions in blood flow through the retinal or choroidal circulation, which produce milder hypoxia, play a role in diabetes, glaucoma, and age-related macular degeneration. In diabetes, for example, hypoxia could be caused by the upregulation of adhesion molecules and leukostasis that is known to occur. In many tissues, hypoxia leads to a dramatic elevation in the level of the transcription factor hypoxia-inducible factor 1α (HIF-1α), not because synthesis is increased, but because degradation is prevented during hypoxia. HIF-1α increases in the retina in many situations. HIF-1α has multiple downstream effects, including the upregulation of vascular endothelial growth factor (VEGF), which is a hallmark of diabetic retinopathy and wet macular degeneration. Complicating the picture, however, are findings that VEGF and HIF-1α can increase as a result of factors other than hypoxia.
The second role for oxygen in disease is the situation where there is nothing wrong with the circulation per se, but there is a mismatch between oxygen supply and metabolic demand. An important and underappreciated example of this is in retinal detachment ( Chapter 71 ), where the choroid is functional, but is too far from the photoreceptors to supply their needs fully. Theoretically, metabolic starvation of the inner-segment mitochondria would occur with even very small separations between the choroid and photoreceptors, as would occur in the presence of large drusen, so it is possible that a supply–demand mismatch contributes to the loss of photoreceptors in dry age-related macular degeneration, which occurs directly over drusen. In these cases the mismatch is caused by insufficient supply. Only one case is known at present where the mismatch is caused by increased metabolic rate. This is the situation that leads to the loss of photoreceptors in some hereditary degenerations ( Chapter 76 ). In rd mice and in some cases of human retinitis pigmentosa, phosphodiesterase (PDE) activity is reduced. This is expected to lead to increased cyclic guanosine monophosphate (cGMP) levels, which in turn would lead to increased numbers of open channels in the photoreceptors, increased influx of Na + and Ca 2+ , and a greater demand for adenosine triphosphate (ATP) to operate Na + and Ca 2+ pumps than the cell can meet. Whether metabolic overload causes loss of photoreceptors or other retinal neurons in other situations is not known.
The third situation is one in which there is too much oxygen. This has long been recognized as a fundamental problem in retinopathy of prematurity ( Chapter 72 ), preventing the growth of retinal blood vessels in the neonate, and more recently it has been recognized to be the reason why retinal blood vessels ultimately disappear or are seri ously attenuated in all forms of retinitis pigmentosa. Oxidative damage is also thought to play a role in reperfusion injury in the retina.
The presence of the choroidal circulation offers possibilties for therapy, because it is rarely compromised at the same time as the retinal circulation. The leading hypothesis for the success of panretinal photocoagulation in proliferative diabetic retinopathy is that laser destruction of the photoreceptors removes their oxygen demand, allowing choroidal oxygen to reach the inner retina. This relieves hypoxia and reduces angiogenesis. Intraretinal P o 2 measurements show that O 2 levels do increase after photocoagulation. Choroidal oxygen is probably also responsible for the benefit of hyperbaric oxygen in cystoid macular edema. We and others have also argued that treatment with hyperoxia would be beneficial in arterial occlusion and retinal detachment, and that attempts at using hyperoxia previously have misunderstood some of the key features of retinal oxygenation outlined below.
This chapter gives an overview of retinal energy metabolism and substrate supply, providing a basis for understanding the potential roles of these topics in the etiology of the diseases covered in more detail elsewhere.
Pathology
There are many studies showing detrimental effects of reduced oxygen and substrates in acute experiments, but only a few studies investigating whether reduced supply of substrates, in the absence of any disease, can cause retinal pathology, because chronic, moderate changes that would mimic disease are more difficult in an experimental setting. However, the experiments that have been done reveal aspects of pathology similar to those that would be observed in disease. For example, in birds, it has been shown that reduced choroidal blood flow can cause photoreceptor loss and upregulation of GFAP, as well as decreased acuity. In rat retina, chronic systemic hypoxia (10% inspired oxygen) leads to apoptosis of photoreceptors. Hypoxia can also itself lead to neovascularization.
Pathophysiology
A number of properties make the retina unique, in terms of: (1) the supply of oxygen and glucose to the retina by its circulations; (2) the utilization of the substrates to provide ATP; and (3) the relative importance of the cellular processes that use the ATP. A great deal is known about the first two topics. The third has been more difficult to investigate and our knowledge is mainly about photoreceptors.
Supply of oxygen and glucose from the circulation ( Box 73.2 )
The retina of humans and many other mammals is supplied by two circulations, both of which are essential ( Figure 73.1 ). The choroidal circulation lies behind the retinal pigment epithelium and the retinal circulation lies within the inner half of the retina, except in the central part of the fovea, where the retinal circulation is absent. Because the two circulations have such different physiological properties, they must be treated separately. The retinal circulation is like the circulation of the brain in most respects. The flow rate is modest, on the order of 25 µl/min, or 20–25 ml/(100 g-min) of retina if normalized to the whole retinal weight, or about 40–50 ml/(100 g-min) if normalized by the inner retinal weight (half the total retinal weight), which is what the retinal circulation normally supplies. This is called the “nutrient flow rate” below. The retinal blood flow rate is controlled by tissue oxygen and metabolite levels, with hypoxia and hypercapnia increasing flow, and hyperoxia decreasing flow. Increased activity of retinal neurons caused by visual stimuli can increase retinal blood flow, but the blood flow in steady illumination is only transiently increased relative to the flow in the dark. There is no autonomic control of the retinal vessels after the retinal artery enters the eye at the center of the optic nerve.
Retinal
- •
Modest flow rate (40–50 ml blood/100 g tissue/min)
- •
High oxygen extraction (venous saturation 8 vol% below arterial saturation)
- •
Metabolic local control by neural activity, tissue P o 2 , tissue CO 2 , pH, and lactate
- •
Good autoregulation in the face of changing perfusion pressure
- •
Microcirculation similar to that of brain, but more pericytes in capillary walls
Choroidal
- •
Unusually high flow rate (1200 ml blood/100 g tissue/min)
- •
Low oxygen extraction (venous saturation 1 vol% below arterial saturation)
- •
Control exclusively by autonomic system
- •
Modest ability to autoregulate in the face of pressure changes
- •
Microcirculation with large fenestrated capillaries

In contrast, the choroidal circulation has a flow rate of 700–900 µl/min, or 1200–1500 ml/(100 g-min) if normalized by choroidal weight, or still slightly higher if normalized by the outer retinal weight. The choroidal flow rate changes little in response to hypoxia or hyperoxia, although it does increase in hypercapnia. Instead, autonomic control is very strong, with sympathetic influences decreasing flow, and facial nerve stimulation increasing flow. Flow rate increases somewhat in response to illumination, but this is when the photoreceptors need less oxygen, so it cannot be a metabolically dependent effect.
Oxygen supply
The retina is critically dependent upon a continuous supply of oxygen, with loss of vision occuring only 5–10 seconds after intraocular pressure is raised to a level that occludes all circulation to the retina. It is known that oxygen is the important factor, because vision can be slightly, but not usefully, prolonged if an individual breathes oxygen before the occlusion. Levels of oxygen in the retina have been measured by a variety of techniques, including optical and magnetic resonance measurements that are valuable because they are noninvasive, but here we focus on microelectrode measurements within the retina and measurements of oxygen in the two circulations, which yield information useful for determining metabolic rate.
The choroidal circulation is normally responsible for most of the oxygen needed by the photoreceptors, while the retinal circulation provides most or all of the oxygen needed by the inner retina. Both circulations are required, and neither can compensate for the loss of the other under ordinary conditions. One might expect that there would be a minimum P o 2 at the depth where the supply from the two circulations meets. There is such a location, but it varies with illumination, and may be different in different animals. In darkness in cat, monkey, and presumably human retina, the minimum P o 2 is not at the middle of the retina, but is far distal, in the photoreceptor inner-segment layer, about 35 µm from the choroid ( Figure 73.2 ). Despite the distal location of this minimum, the retinal circulation only pro vides about 10% of the oxygen used by the photoreceptors. During strong illumination, in cat and rat, the oxygen gradient extends further toward the inner retina, and generally provides all of the oxygen for the photoreceptors, and possibly a little for the outer plexiform layer. However, in monkey, the change in the shape of the gradient is less dramatic, and the choroid does not provide all the oxygen for the photoreceptors during illumination.

One of the often confusing aspects of retinal oxygenation is why choroidal blood flow should be so high. By employing the Fick principle, a mass balance on oxygen, it is possible to calculate the total amount of oxygen extracted from each circulation:
Q O 2 c = F c ( S a O 2 − S v c O 2 ) for the choroidal circulation , and
Q O 2 r = F r ( S a O 2 − S v r O 2 ) for the retinal circulation .
Q o 2 c = 1 4 0 0 × ( 0 . 2 − 0 . 1 9 5 ) = 7 . 0 0 m l O 2 / ( 1 0 0 g m i n )
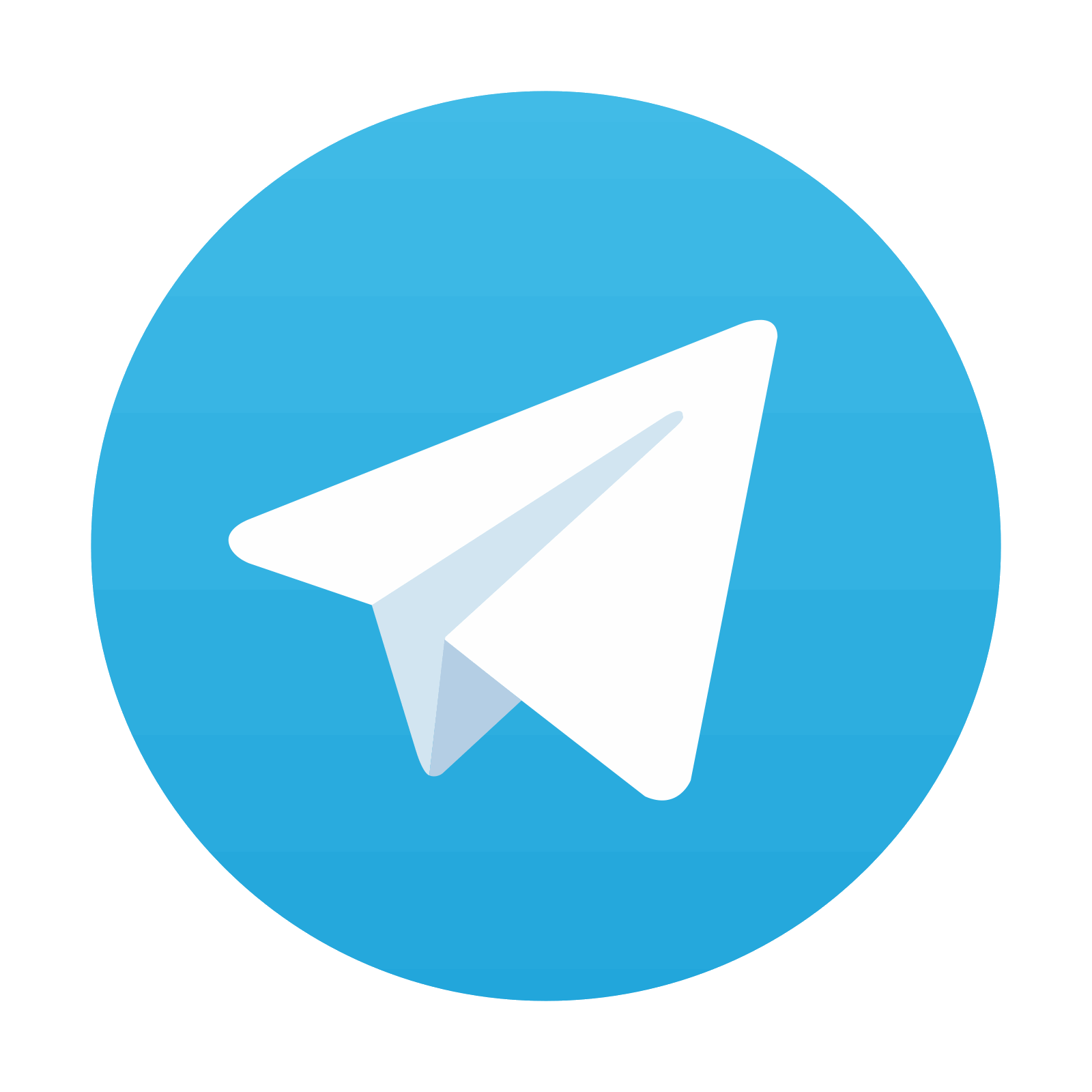
Stay updated, free articles. Join our Telegram channel
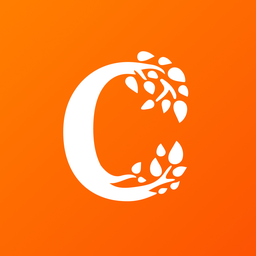
Full access? Get Clinical Tree
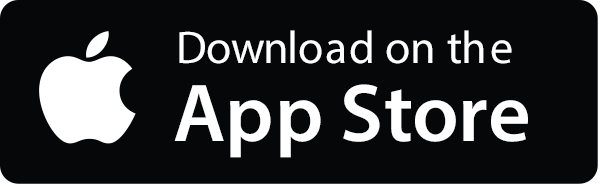
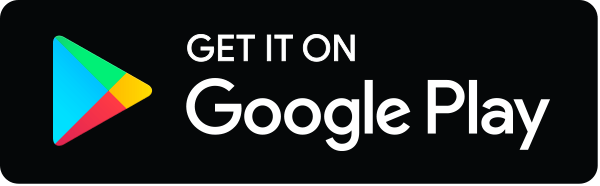