32
Radiotherapy Treatment for Age-Related Macular Degeneration
Raul Velez-Montoya
Virgilio Morales-Canton
Hugo Quiroz-Mercado
INTRODUCTION
Age-related macular degeneration (AMD) is a complex disease and difficult to define. Since its first description by Holloway and Verhoeff in 1929 as a condition characterized by the loss of vision in older patients due to degenerative changes in the retina pigment epithelium (RPE) (1), the amount of knowledge about this disease, as well as the interest in finding an adequate therapy, has been increasing exponentially. Eighty years later, despite all the resources and efforts put forth to better understand the etiology and pathophysiology of AMD, many of its mechanisms remain elusive.
As life expectancy in developed countries and around the world has increased, AMD incidence has also risen to become the leading cause of vision loss among individuals aged 50 years or older (2–4). It is believed that the annual incidence of some degree of AMD among patients 60 years or older reaches 40% to 50%. AMD-related disability and poor quality of life have become heavy socioeconomic burdens in industrialized countries (5–7).
Although most AMD patients suffer the atrophic or “dry” form, the neovascular or “wet” form will be found in more than 10% of the cases. Wet AMD is characterized by deep choroidal neovascularization (CNV), subretinal fluid (SRF), subretinal hemorrhage (SRH), exudates, scarring, and severe visual loss (8–11). The natural history of the disease dictates that approximately 75% of the patients with subfoveal exudative lesions will become legally blind after 2 years (12, 13). Population studies such as the Beaver Dam Eye Study demonstrated that approximately 10% to 20% of patients with the dry form of AMD will progress to the exudative form (especially if the patient’s retina exhibits large drusen [≥125 μm], soft indistinct drusen, retinal pigment epithelium abnormalities, or exudative and geographic lesions). This phenomenon of dry to wet progression was likely responsible for most of the estimated 1.2 million cases of visual loss due to AMD (14–16) which occurred in the 1990s in the United States and may lead to 7 million by the year 2025, given that 4% of the wet AMD cases are classified as severe (17) (Fig. 32-1).
Although the etiology of the AMD is still largely unknown, there has been an improvement in understanding the pathophysiology of the disease. In a normal eye, the byproducts of photoreceptor metabolism are usually removed by the RPE cells (18). It is believed that the aging process slows down RPE metabolism, diminishing its phagocytosis and lysosomal capacity, and resulting in accumulation of cell debris, clinically seen as drusen (5, 18, 19). Over time, these changes gradually thicken Bruch’s membrane and make it more hydrophobic, impairing oxygen delivery to photoreceptors (19–21). It is not clear why this aging process ends in cell loss and atrophy in dry AMD, while in the wet form it stimulates a choroidal neovascular response. What we do know is that the hypoxia induces a low-grade inflammatory cascade which ends in the production of several growth factors, including vascular endothelial growth factor (VEGF), found to have a key role in CNV pathogenesis (2, 22). VEGF is a dimeric lipoprotein whose principal biological activity is to produce an increase in the vascularization of hypoxic tissues (23, 24). This is accomplished through several mechanisms: increase in the mitotic activity of vascular endothelial cells; increase in vascular permeability via leukocyte-mediated endothelial cell injury, formation of fenestrae and dissolution of tight junctions; and induction of activated endothelial cells to produce enzymes and metalloproteinases which digest the capillaries’ basement membrane, the surrounding ground substance, and Bruch’s membrane, thus forming spaces and breaks through which newly formed vessels pass into the subretinal space (2, 18, 23–26).
Figure 32-1. Color picture (A), fluorescein angiography (B and C), and spectral domain optical coherence tomography (D) of a 65-year-old female with clinical diagnosis of wet age-related macular degeneration. The color picture shows the presence of subretinal fibrosis and subretinal hemorrhage. B and C show middle and late fluorescein leakage, which indicates an active lesion. D shows the presence of a subretinal hyperreflective lesion (fibrosis) and hyperreflective spaces, which may indicate the presence of blood or subretinal fluid.
It has also been demonstrated by histological studies that a granulomatous inflammatory response to the degenerated Bruch’s membrane is additionally responsible for the formation of breaks (23–30). This is supported by the observation of lymphocyte and macrophage infiltration of Bruch’s membrane and accumulation of macrophages at the site of neovascularization in some animal models of laser-induced CNV (23–30). However, it is still unknown whether this inflammatory response is part of the aging process of the eye or if the macrophage and lymphocyte activation is necessary for the CNV process (23).
Once the CNV reaches the subretinal space, the visual impairment will be closely related to the lesion size, the amount of exudates, and thickness of the disciform fibrosis. Any therapeutic approach that helps to control or reduce the three pathways of VEGF action described earlier may result in the stabilization or improvement of visual acuity in patients with wet AMD (31, 32).
Basics About Radiation Therapy for AMD
The application of radiation to treat AMD is based on the concept that CNV formation is analogous to a wound-healing process, where the high rate of proliferating endothelial cells makes them more sensitive to the effects of radiation (33–35). Reinhold described that a single dose of 8.7 Gy on normal capillaries leads within hours to swelling and vacuolation of the endothelium cells’ cytoplasm and local vasodilation (8, 36). Reinhold also describes that the radiation effects continue through time, until the loss of endothelial nuclei occurs and a reduction is seen in the number and length of the capillaries and occlusive changes (8, 36, 37). The clinical effect of radiation on the eye vessels was first described in plaque-irradiated choroidal melanoma patients where, following plaque removal, a ring of choroidal atrophy with decreased or absent blood flow by fluorescein angiography (FA) was observed around the tumor’s base (18, 38). A similar response has also been seen in choroidal hemangiomas and intracerebral arteriovenous malformations, where radiation is used to induce regression, and in ocular and cutaneous wounds, where radiation stunts the growth of newly formed vessels without thermal injury associated with lasers and cautery (8, 18, 34, 39–41).
Although the biological justification to use radiation to treat CNV seems clear, there is controversy regarding the exact mechanism that drives the radio effect (42). The use of ionizing radiation induces the formation of free radicals (mainly from water molecules), which in turn cause irreparable damage to the DNA backbone and disrupt protein synthesis. The irradiated cell loses its ability to replicate and migrate but does not lose its cellular integrity nor undergoes necrosis (33–35). On the other hand, there is evidence that radiation induces programmed cell death in vivo (33&x2013;35, 43, 44). Miyamoto et al. studied the histologic appearance of rabbit eyes with experimental CNV, 4 weeks after a single fraction exposure to 20 Gy of focal X-ray irradiation (45, 46). The degree of vascular formation and the number of endothelial cells in the CNV of irradiated eyes were less than in those of control eyes. And although the pathogenesis of experimental CNV and CNV due to AMD are not identical (45, 46), the selective toxicity of radiation on rapidly dividing endothelial cells seems clear.
The use of sublethal radiation doses, low enough to spare cell life but high enough to induce DNA damage, can produce growth arrest by altering the genes of endothelial-growth–regulating cytokines and genes of inflammatory cytokines interactively affecting different cell populations (8, 47, 48). In vitro investigations of radiated endothelial cells showed an upregulation of basal intracellular adhesion molecule-1, which participates in the radiation-induced inflammatory reaction of the endothelium, facilitating inflammatory cells adhesion (49). All these actions decrease the CNV growth profile and the inflammatory response, which is thought by many to play a role in the formation of CNV (18, 50, 51).
Although radiation effects on endothelial cells are important in its ability to impact CNV, the power of radiation to affect inflammatory cells and fibroblasts is also a key factor, as it alters the formation and deposition of collagen, ultimately inhibiting scarring and fibrosis, which are important components of end-stage AMD (32, 39, 40, 52). The results of the Belfast Study Group, showing that deterioration of visual acuity is less marked and scars are smaller in irradiated eyes than in untreated eyes at 6 to 24 months of follow-up, and that there is a positive correlation between scar size and better visual acuity, seem to support this assertion (53, 54). Radiation reduces the expression of basic fibroblast growth factor and transforming growth factor β1, as well as the synthesis of prostacyclin (32, 55), which decreases fibroblast proliferation and collagen deposition in vitro, and may play a role in reshaping the disciform scars in radiated eyes (32).
Despite the fact that radiation is potentially damaging to the retina, optic nerve, and the lacrimal system, the most frequently described adverse effects of clinical radiation in the eye are epiphora and transient conjunctival irritation (14). The rod photoreceptors are the most radiosensitive cells in the retina; cell death, however, is not seen at doses less than 10 Gy and RPE cell loss does not occur at less than 20 Gy (14). Radiation retinopathy is unlikely to occur at doses of 25 Gy or less, especially if delivered in fractions and/or over small volumes of the retina (8, 56–58). But even though the retina and REP are the most radiosensitive tissue of the eye (59), it is the lens that is the most sensitive to long-term exposure. Radiation damage to the lens occurs on two levels: acute radical reactions involving lens crystallins or enzymes of the carbohydrate metabolism and the oxidative defense system, leading to transient or definitive transparency changes, and radiation-induced damage to DNA of lens epithelial cells (14, 59–62).
We can therefore speculate that there is a therapeutic window where radiation can spare normal tissue but yet have a selective toxicity over the endothelium, fibroblasts, and inflammatory cascade, targeting specific pathways that pharmacologic anti-VEGF therapy does not presently reach by itself (18).
EARLY EXPERIENCE WITH RADIATION THERAPY FOR AMD
For over 30 years, studies investigating the use of radiotherapy in the treatment of AMD have shown mixed results. To recount every single published paper on the subject would be impractical, if not tedious. Since many of these publications addressed case series, uncontrolled small studies, and other less reliable data, only the most relevant (in the authors’ opinion) will be mentioned here. The general treatment options consisted of two broad strategies: (a) teletherapy (delivering a beam of radiation over a distance to the patient) using external beam, proton beam, charged particles, or gamma-knife and (b) plaque brachytherapy using radioactive isotopes (I-125, Palladium-103 [Pd-103], Strontium-90 [Sr-90], ruthenium [Rhu]) (Fig. 32-2). Broadly speaking, plaque brachytherapy was superior to teletherapy with respect to targeting and complications. This is primarily because the brachytherapy plaque was sewn onto the sclera directly over the macula. The treatment volume was constrained to a limited region, and the optic nerve and nonmacular retina received much less energy than with teletherapy, unless, of course, the plaque became dislodged over the course of the several-day–long treatment. The obvious drawback to plaque brachytherapy was that, due to its position immediately over the macula, the plaque (and the radiation) was directly aimed at the lens. For all intents and purposes, this meant that every phakic patient developed a cataract shortly after therapy. The second drawback was that it required two surgeries—one to place the radioactive plaque and the second to remove it. These surgeries often necessitated disinsertion of one or more muscles, and double vision was not an uncommon complication. Of course, the development of a cataract often required yet another operation to replace the lens.
Experience with Plaque Brachytherapy
The first isotope employed in a pilot study for the treatment of wet AMD using plaque brachytherapy was Pd-103 (63). In this first study, Finger et al. treated six patients with 12-15 Gy, resulting in a decrease in hemorrhage, exudates, and leakage on FA, while sparing the neurosensory retina, with no evidence of radiation retinopathy or optic neuropathy (63). Based on the positive result of this preliminary study, a pilot open label, uncontrolled, nonrandomized phase I study was designed, where 23 patients received Pd-103 plaque brachytherapy for the treatment of wet AMD, with a dose ranging from 12.5 to 23.62 Gy (64). The mean follow-up was 19 ± 10.7 months. In this second study, a moderate visual acuity loss (≤3 ETDRS lines) was seen in 16% of subjects at 6 months, 31% at 12 months, and 22% at 24 months of follow-up (64). For data analysis, the funduscopic and angiographic findings were grouped in the following categories: (a) improved lesions—decreased or resolved hemorrhage, exudates, and/or fluid; (b) stable lesions—relatively unchanged or minimal enlargement; and (c) progressive—increased lesion size (64). For all lesions at last follow-up, there was an 87% rate of stable or improved funduscopic and angiographic findings, but approximately 30% of the cases developed recurrent lesions (64). The rate of progression at 19 months was 9%, and 61% were stable or improved (64). There were no reported cases of radiation retinopathy, optic nerve injury, or complications related to the surgical technique. The same authors, in a separate report (2003), described the outcomes of a 7-year follow-up of 31 eyes treated with Pd-103 brachytherapy (65). The mean radiation dose was 17.25 Gy (range 12.5 to 24 Gy) in a single fraction. The visual acuity results at last follow-up (mean 33.3 months, range 3 to 84 months) included loss of ξ3 ETDRS lines at 55%; improvement of ξ3 ETDRS lines at 16%; and within 2 ETDRS lines from baseline at 29% (65). The most common anatomic outcome (69%) was regression or stabilization of the exudative process. The authors reported a rate of severe visual loss (ξ6 ETDRS lines) at 6 months in 4% of the subjects, which progressed to 5% at 12 months and 25% at 48 months. At last follow-up, no patient had developed radiation retinopathy, optic neuropathy, or cataract (65).
Another isotope which has also been extensively explored as plaque brachytherapy is Sr-90 (66). In a study carried out by Jaakkola et al. in 1998, 20 patients received a 54-minute application of Sr-90 to achieve a dose of 15 Gy. The visual acuity was within 5 letters of baseline or improved in 55% of patients, and 74% of patients demonstrated either a decrease in CNV size or a decrease in the amount of late leakage on FA (66). At 12 months of follow-up, 74% of patients demonstrated partial or total occlusion of the CNV (66). Two years later, the same authors published a larger randomized controlled clinical study which appeared to include the previous 20 patients (67). On this occasion, 86 patients divided into a study (43) and a control (43) group were included. The study group was again divided into two subgroups. The first one (18 patients) received a 54-minute therapy consisting of 15 Gy, and the second one (25 patients) received an 11-minute therapy consisting of 12.6 Gy (67). During the first 12 months of the study, the treated group had a significantly lesser risk of moderate visual loss (>15 ETDRS letters), but that advantage diminished by 24 and 36 months of follow-up. The 15-Gy dose seemed to be superior to the 12.6 Gy in terms of moderate visual acuity loss (67). The FA outcomes demonstrated statistically significantly less lesion activity with respect to baseline at the 6-month follow-up in 37.5% of the patients and 56.4% at the 12-month follow-up, favoring the study group (67).
Figure 32-2. Treatment plan and schematization of teletherapy for AMD. A: Dose distribution and treatment plan of a 16-MV electron beam of 4 × 4 cm2. The macula is enclosed by the 100% isodose, while the rest of the orbit also receives radiation. B: Computer tomography with superimposed treatment plan showing the isodose distribution for 6-MV photon beam. The green circle shows the 95% isodose, focused on the fovea. (Part A from Lambooij AC, Kuijpers RW, Mooy CM, et al. Radiotherapy of exudative age-related macular degeneration; a clinical and pathologic study. Graefes Arch Clin Exp Ophthalmol 2001;239:539–543. Part B from Eter N, Wegener A, Schüller H, et al. Radiotherapy for age related macular degeneration causes transient lens transparency changes. Br J Ophthalmol 2000;84:757–760.)
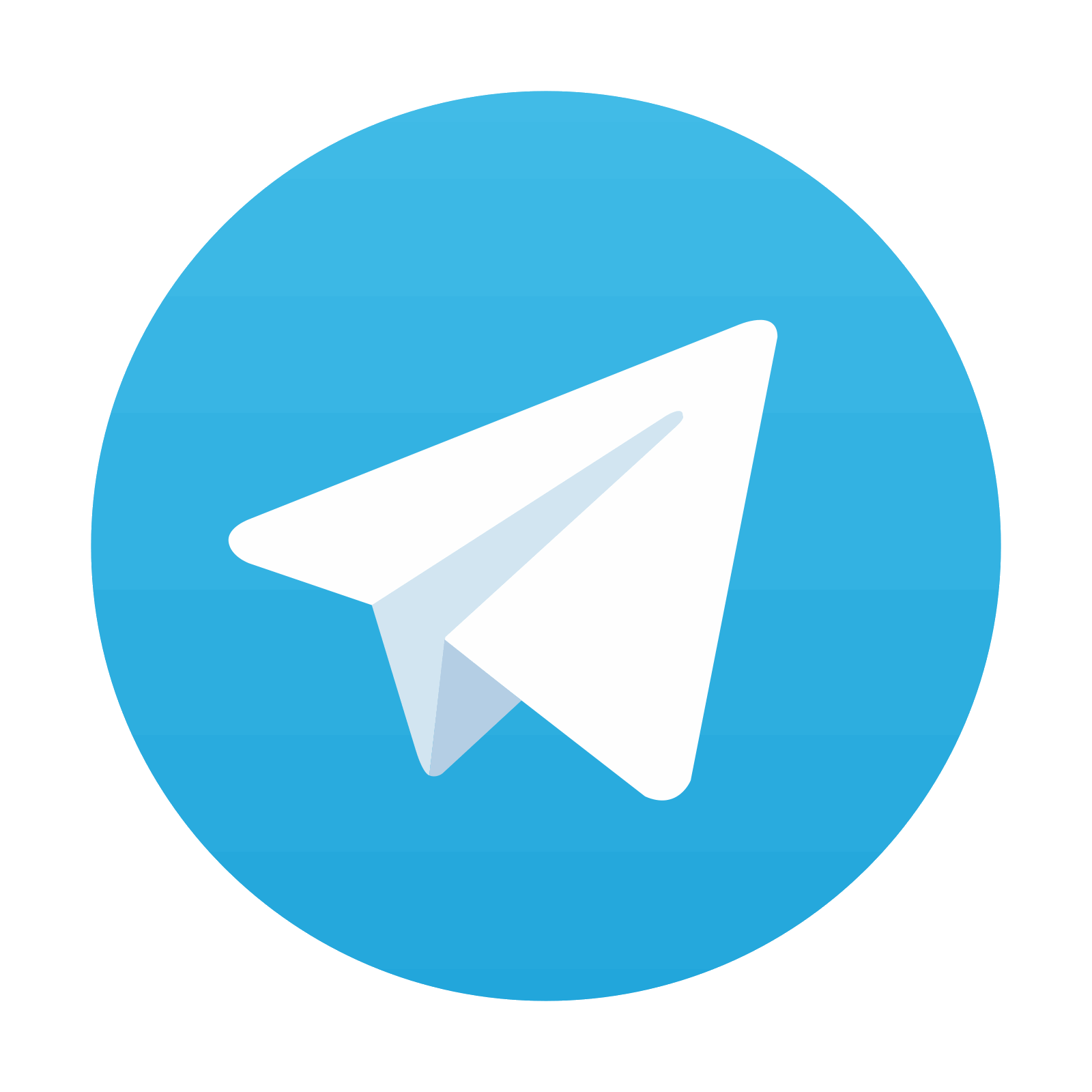
Stay updated, free articles. Join our Telegram channel
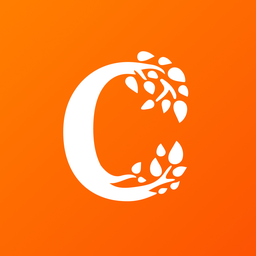
Full access? Get Clinical Tree
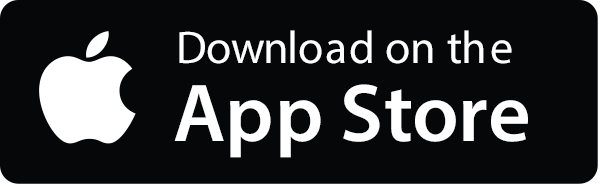
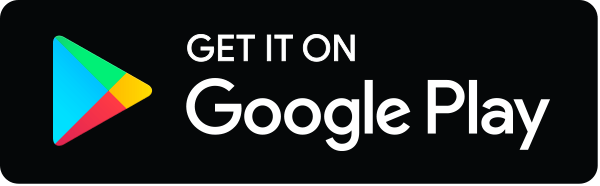