Fig. 31.1
Establishment of pluripotent stem cells. (a) ES cells are derived from inner cell mass. (b) iPS cells are derived from somatic cells, using viral transfection, plasmid transfection, mRNA transfection, recombinant protein introduction, and small-molecule introduction, followed by selection. (Modified from Narsinh et al. [1])
These unique cells are used in many research aspects. One big achievement in the ESC study is the generation of the genetically modified animals. The genome of ESC can be modified by random mutagenesis, homologous recombination, or gene insertion (transgene). When ESCs are introduced into another blastocyst, the resulting animal, which is called chimera, consists of two kinds of cells, one derived from the original inner cell mass and another from the exogenous ESCs. If the ESC contributes to the germ cells, this animal is called germ-line chimera, and this phenomenon is called germ-line transmission [12]. All the cells in the animal that is derived from ESC germ cells have the same genome as the ESC. By this strategy, ESCs are widely used for the generation of knock-out/knock-in animals, chimeras, and clones. Nowadays, these genetically modified animals are indispensable tools for biological research and animal industry.
Another achievement of ESC-related research is the investigation on the “stemness”: essential characteristics of a stem cell that distinguishes it from differentiated cells. Several factors that are related to the stemness have been identified through the study of ESCs [13]. Among them, Oct3/4, Sox2, c-Myc, and Klf4, which are predominantly expressed in ESCs, were identified as the sufficient set of factors to reprogram somatic cells into ESC-like character, named iPSCs [14].
The most challenging part of ESC study is the understanding mechanisms of differentiation and controlling it. ESCs derived from the inner cell mass are a good in vitro model for the study of cellular differentiation in early embryogenesis. Extending this study, ESCs were induced to differentiate into a wide variety of cell types including hepatocytes, pancreatic islet cells, blood cells, chondrocytes, cardiomyocytes, dopaminergic neurons, Schwann cells, retinal pigmented epithelium, and so on, and expected to be applied in clinics [15].
31.2.2 Induced Pluripotent Stem Cells (iPSCs)
iPSCs are somatic cell-derived stem cells similar to ESCs in terms of self-renewal and pluripotency. iPSCs were first established by Takahashi and Yamanaka in 2006 [14] (Fig. 31.1). They introduced Oct3/4, Sox2, Klf4, and c–Myc using retrovirus vector into mouse embryonic fibroblasts and selected using the Fbx15 reporter system, which is specifically expressed in mouse ESCs and early embryos, but is dispensable for the maintenance of pluripotency and mouse development. Resulting cells were similar to mouse ESCs in many points such as an expression of stem cell-specific genes and surface glycoproteins, chromatin methylation patterns, and differentiation potency into three germ layers in vivo and in vitro. However, these cells were not able to contribute to adult chimeras. To improve the quality as pluripotent cells, fully reprogrammed cells were selected by the expression of Nanog, which is required for maintaining pluripotency, and germ-line chimeras were obtained as a result [16]. In the next step, human iPSCs were established by using the same set [17] or another set of transcription factors (OCT3/4, SOX2, NANOG, LIN28) [18]. These human iPSCs also fulfilled the same criteria as mouse iPSCs and ESCs, except for confirmation of chimera formation and germ-line transmission, which is obviously ethically unacceptable.
After the generation of iPSCs from fibroblasts [17], iPSCs have been generated from various types of cells to demonstrate a proof of principle that all somatic cells can be reprogrammed into pluripotency: murine liver and stomach cells [19], pancreatic β-cells [20], terminally differentiated lymphocytes [21], cord blood cells [22], third molar [23], keratinocytes of plucked hair [24], and neural stem cells (NS cells) [25, 26]. iPSCs were generated also from cochlear epithelial cell-derived otospheres of neonatal mice [27]. Although otospheres resemble multipotent NS cells in their overall gene expression pattern and epigenetic status [28], the reprogramming efficacy of NS cells by four factors was approximately 10 times higher than that of otospheres [25, 27]. Differences in demethylated patterns of NS cell-specific Sox2 enhancers may partly cause the difference in reprogramming efficacy.
More recently, it was reported that less factors are required to reprogram somatic cells into pluripotency. iPSCs were generated from mouse and human fibroblasts without Myc [29] and from keratinocyte and mesenchymal stem cells by three factors: OCT3/4, SOX2, and KLF4 except c–MYC [30, 31]. Additionally, iPSCs were generated from human NS cells by introduction of OCT3/4 and KLF4, or only OCT3/4, suggesting that endogenous expression of the reprogramming factors can complement exogenous factors [32].
31.2.3 Disease-Specific iPSCs
The genetic mutation in a genome of a patient is taken over to iPSCs, when somatic cells of the patient are reprogrammed to iPSCs. These cells, so-called disease-specific iPSCs, provide opportunities to develop novel strategies to elucidate the pathological mechanisms of disease, together with physiological mechanisms by comparing with wild-type cells. Differentiated cells from disease-specific iPSCs into the target or precursor cells serve as in vitro disease models, that is, recapitulation of the disease phenotype at cellular level, and are used to reveal molecular mechanisms of the disease. These differentiated cells are also useful to develop new treatments. Soon after the establishment of human iPSCs [17], mutant iPSCs were successfully generated from several diseases including amyotrophic lateral sclerosis (ALS) [33], muscular dystrophy, Parkinson disease, and Huntington disease [34], followed by the research for cell survival and function of spinal muscular atrophy patient iPSC-derived motor neurons [35]. Since then, disease-specific iPSCs have already been generated to establish disease models, from neurological disorders [36–46], cardiovascular diseases [47–51], metabolic abnormalities [36, 52, 53], and hematological insufficiency [54–56].
The major advantage of the use of disease-specific iPSCs owes much to the ability to generate a specific tissue/cell type from the cells obtained from another tissue. For example, the pathogenetic mechanism in Alzheimer’s disease (AD) comes from chronic accumulation of abnormal amyloid-β peptide (Aβ) oligomers in the brain tissue. However, brain biopsy for the diagnosis or research is not easy, and, before the time of diagnosis of AD, neural tissue has been extensively modified due to the chronic accumulation of Aβ. By using several lines of iPSCs derived from AD patient’s skin fibroblasts (AD-iPSC), Kondo et al. [42] induced AD-iPSC-derived neurons. They successfully detected stress responses as an early cellular phenotype and showed that docosahexaenoic acid (DHA) treatment alleviated the stress responses in the AD-iPSC-derived neurons from a subset of patients. This not only clarified the early mechanism of the disease, but also may help select patients who are responsive to DHA treatment. iPSCs derived from skin fibroblasts of dilated cardiomyopathy (DCM) patients [50] also provided a good opportunity to perform cellular study, because cardiac tissues from DCM patients are difficult to obtain and do not survive in long-term culture.
Disease- or patient-specific iPSCs are used for drug screenings in vitro to identify candidate chemical compounds for the therapeutic purpose [39, 40, 42, 50, 57] or to test toxicity of drugs [48, 51]. For example, a histone acetyltransferase inhibitor called anacardic acid was identified that rescued the phenotype of abnormal motor neuron differentiated from ALS-iPSCs [39].
Mutations in mitochondrial DNA (mtDNA) are implicated in numerous human diseases. Artificial modification of mtDNA would be one possible strategy to treat diseases due to mutations in mtDNA. However, the modification of mtDNA is difficult because mtDNA is encapsulated in the mitochondrial outer and inner membrane, and multiple copies of mtDNA exist in each cytoplasm. Mitochondria in each somatic cell are maintained during the process of reprogramming for the generation of iPSCs. Thus, resulting iPSCs that carry the mutant mtDNA serve as an in vitro model for these diseases. Disease-specific iPSCs have been established from those patients including mitochondrial encephalomyopathy with lactic acidosis and stroke-like episodes (MELAS) [58, 59] and Pearson marrow-pancreas syndrome [60].
As genomic DNA of iPSCs (and ESCs) has an accessible chromatin state, genomic modification is relatively easier compared to other somatic cells. Thus, recent precision gene modification techniques such as the helper-dependent adenoviral vector [61], zinc-finger nucleases (ZFN) [62], transcription activator-like effector nucleases (TALEN) [63], and CRISPR-Cas9 system [64, 65] are suitable to be applied to modify a genome of pluripotent stem cells. For example, ZFN was used to introduce XIST (the intrinsic X chromosome-inactivating gene) into a trisomic chromosome 21 in Down’s syndrome patient-derived iPSCs, followed by successful trisomy silencing [66]. These techniques will provide a new therapeutic concept of those intractable diseases in the future.
As described above, disease-specific iPSCs are remarkable powerful tools and resources for both research and therapy. The inner ear tissues of a living human are difficult to obtain because the inner ear is encapsulated in the hard bone, and the puncture to that capsule for biopsy results in the serious deterioration of the inner ear function. Furthermore, the amount of the inner ear tissue obtained from the biopsy would be limited and it would be difficult to maintain the obtained tissues in a long-term culture. Disease-specific iPSC technologies will be powerful tools to establish inner ear disease models for the investigation of the pathogenic mechanisms, drug discovery, and invention of new treatment strategies. A wide variety of diseases related to inner ear dysfunction of monogenic, chromosomal, or mitochondrial etiology will be the target of entities that are modeled by patient-specific iPSCs.
31.2.4 “Can iPSCs Replace ESCs?”
There are several advantages of iPSCs over ESCs for research and future clinical applications [1]. The first point is the ethics. The generation of ESCs is ethically problematic because it usually accompanies with the destruction of embryos, which are continuous to the living individual. Some researchers justify the generation of human ESCs because they are generated from the fertilized eggs that are destined to be discarded after in vitro fertilization. For those who do not accept this justification, the generation of ESCs from blastomeres [67] or from preimplanted morula [67, 68] might be acceptable because it does not involve the activity of destroying the whole embryos. However, there is no consensus on propriety of intervening embryos to generate ESCs. On the other hand, iPSCs do not raise this ethical problem because they are derived from somatic cells. Secondly, disease-specific iPSCs can be easily obtained from patients as mentioned above. Theoretically, ESCs generated by nuclear transfer (ntESCs) [69, 70] enable the generation of pluripotent stem cells that code genomic information of transferred nucleus. However, it is costly to prepare for every ntESC, where the nucleus originates from each patient. Thirdly, the premise that iPSCs and their progeny would not cause any immune response in the host due to self-tolerance was supported by two reports that syngeneic iPS-derived cells did not elicit immune responses [71, 72]. But there is an opposing report that teratomas from syngeneic murine iPSCs were immunorejected by a host, whereas those from mouse ESCs were immunotolerant [73]. Kaneko and Yamanaka rebutted this report maintaining that immunorejection of syngeneic iPSCs may have been caused by gene integration [74].
However, human ESC research is still important because there is a serious concern about the stem cell-related malignancy. The generation of iPSCs originally accompanied with gene integration. Such genomic modification is a risk factor for insertional mutagenesis, which may cause malignant cell transformation [75]. To reduce this risk, non-integrating gene delivery methods for the expression of defined pluripotent factors have been reported (Fig. 31.1): iPSCs generated with adenoviruses [76], sendai viruses [77], plasmid vectors [78], removable transposon systems [79], direct delivery of recombinant reprogramming proteins [80], and synthetic modified messenger RNA [81]. The use of a proto-oncogene c-Myc is also a concern, which now can be replaced with L-Myc [82] or eliminated [29]. Genetic defects in iPSCs result in not only tumorigenesis but also differentiation defectiveness. Koyanagi-Aoi et al. [83] identified low-quality iPSC lines out of a relatively large number of human iPSC and ESC lines. iPSC lines of low quality were marked by higher expression levels of several genes expressed from long terminal repeats of specific human endogenous retroviruses and molecular signatures such as RNA expression and DNA methylation patterns. Those iPSCs were prone to form teratoma when neurally induced and transplanted into mouse brains. Thus, human iPSCs have considerable variation in terms of complete differentiation and tumorigenesis.
In terms of quality control, ESCs are still superior, because clinical grade human ESC lines have already been established [84]. Therefore, the use of iPSCs in the clinic will require much more research and understanding, and human ESCs will provide an accurate reference for iPSCs, which will allow them to be standardized for their use in clinical applications.
31.3 The Use of Pluripotent Stem Cells for the Regeneration of the Inner Ear
Since Ito et al. first demonstrated the regeneration of the auditory pathway by transplants of fetal brain tissue [85], several stem cell-based approaches to cure deafness using mesenchymal stem cells, neural stem cells, ESCs, and iPSCs have been adopted. Current major targets are cochlear hair cells and SGNs, which are primarily compromised and unregenerable cells in sensorineural hearing loss.
31.3.1 Specific Induction to the Inner Ear Sensory Fate
Inner ear hair cells of the mammals have very little capacity of regeneration [86, 87]. This is one major reason for the difficulty in recovering from permanent profound hearing loss. One suggested modality for the recovery from the hearing impairment is the regeneration of hair cells. In vitro differentiation of inner ear hair cells from pluripotent stem cells is regarded as an important milestone to realize stem cell-based rescue of inner ear hair cells.
Developmental biology of inner ear has given clues for stepwise differentiation of pluripotent stem cells toward the inner ear sensory fate. Currently, there is no universal method to induce auditory and vestibular sensory cells from pluripotent stem cells at high conversion rates. As many researchers struggled for the in vitro induction of inner ear hair cells from stem cells [88], Li et al. first reported the induction of hair cell-like cells from mouse ESCs [89] (Table 31.1). Mouse ESC-derived embryoid bodies were cultured with EGF and IGF-1 on adhesive culture dishes and then supplemented with bFGF to obtain otic progenitor cells. These cells were further differentiated and became positive for hair cell markers and possessed hair bundle-like F-actin staining at the apical surface.
Table 31.1
Methods for differentiation of ESCs/iPSCs into inner ear hair cells
Authors | Cell source | Method | Specific factors and/or conditions | Methods of testing hair cell identity/quality |
---|---|---|---|---|
Li et al. [89] | Mouse ES cell | EB formation, followed by adherent culture | Serum-free conditions | Hair cell marker expression |
EGF, IGF-1, bFGF | ||||
Oshima et al. [90] | Mouse ES cell | EB formation, followed by adherent culture and coculture with stromal cells | Dkk1, SIS3, IGF-1 | Hair cell marker expression |
bFGF | ||||
Morphology by SEM | ||||
Electrophysiological analysis | ||||
Mouse iPS cell | ||||
Chicken utricle stromal cells | ||||
Chen et al. [91] | Human ES cell | Adherent culture | FGF3, FGF10 | Hair cell marker expression |
Retinoic acid, EGF | ||||
Morphology by SEM | ||||
Electrophysiological analysis | ||||
Koehler et al. [92] | Mouse ES cells | Cell aggregate formation by SFEB method, followed by sequential exposure to differentiation factors | BMP4, TGF-β inhibitor | Hair cell marker expression |
Morphology by TEM | ||||
BMP inhibitor, bFGF | Electrophysiological analysis | |||
FM1-43FX uptake | ||||
Ouji et al. [93] | Mouse ES cells | EB formation, followed by culture in conditioned medium of a stromal cell line cells | Conditioned medium by a stromal cell line cells | Hair cell marker expression |
Morphology by SEM | ||||
FM1-43FX uptake | ||||
Ouji et al. [94] | Mouse ES cells | EB formation, followed by forced expression of Math1 gene | Forced expression of Math1 gene | Hair cell marker expression |
Morphology by SEM | ||||
FM1-43FX uptake |
Oshima et al. improved the stepwise method and tested electrophysiological property of the induced cells [90]. Embryoid bodies from mouse ESCs or iPSCs were cultured with Dkk1 (Wnt inhibitor), SIS3 (selective inhibitor of Smad3 that interferes TGF-β signaling), and IGF-1 to induce rostral ectodermal linage, followed by the treatment with bFGF on adhesive culture dishes to enrich otic population using Pax2 as an otic marker. These cells were cocultured with chicken utricle stromal cells to further differentiate into hair cell-like cells, with the expression of multiple hair cell markers including Atoh1 and Myosin7a, and stereocilia- and kinocilia-like structures. These hair cell-like cells responded to mechanical stimulation with currents that were reminiscent of immature hair cells.
Chen et al. showed that human ESCs also have the capacity to differentiate into hair cell fate [91]. Human ESCs were plated as monolayer and supplemented with FGF3 and FGF10, which are synergistically required to induce otic placode fate in mice. Epithelial cell-like colonies were manually picked up and further differentiated in the media supplemented with all-trans-retinoic acid and EGF. Resulting culture included hair cell marker-positive cells with immature stereocilia-like structures.
Koehler et al. further broke down the early developmental steps and applied to mouse ESCs utilizing serum-free floating culture of embryoid body-like aggregates with quick reaggregation (SFEBq) system [92]. Mouse ESC aggregates were treated with BMP4 and TGF-β inhibitor (SB-431542), and then their outer epithelium showed definitive ectoderm markers (AP2 and Ecad). Next, BMP-/SB-treated aggregates were exposed to BMP inhibitor (LDN-193189) and FGF2, and then thickened patches were formed in the outer epithelium, mimicking the otic placode in terms of morphology and marker expression (Pax8 and Ecad). After further differentiation culture, otic vesicle-like structures are formed in the BMP-/SB-FGF-/LND-treated aggregates. Wnt inhibitor from days 8 to 10 significantly reduced the number of vesicles, indicating the role of endogenous Wnt for the formation of otic vesicles. By day 16, the vesicles were lined with hair cell-like Myo7a(+) Sox2(+) cells with F-actin-rich stereocilia-like structures. Hair cell-like cell layer was tightly arranged with Sox2(+) supporting cell-like cells. The rapid uptake of FM1-43 dye and the diversity of voltage-dependent currents demonstrated that induced hair cells were functional. From the expression of calbindin 2, these hair cells seemed to be equivalent to vestibular type II hair cells.
Approaches above are stepwise differentiation mimicking the embryonic inductive signals. Another approach is the direct induction. Ouji et al. [93] used a conditioned medium by a stromal cell line (ST2) to culture mouse ESC-derived embryoid body, and up to 20 % of outgrowth from the embryoid body expressed several hair cell markers, and its induction rate has been improved by Atoh1 overexpression [94].
As introduced here, hair cell induction from pluripotent stem cells has gradually become a reliable method. This method will directly be applied to patient-specific iPSCs to elucidate the mechanism of inner ear diseases or for the drug screening. However, hurdles still exist before the realization of hair cell regeneration. It is still necessary to improve the induction rate and to develop the method to specifically induce cochlear hair cells. More fundamental difficulty is the in vivo application of induced hair cells. It is not realistic to transplant mature hair cells and to arrange them beautifully in the organ of Corti [95]. Other approaches would be necessary. Introduction of inner ear inducers shown above in a time- and concentration-controlled manner to initiate differentiation of inner ear stem cells, introduction of stem cells which are programmed to differentiate to inner ear sensory fates, and introduction of stem cell-based factor-releasing cells may also be other possible strategies.
31.3.2 Neural Induction and Transplantation
SGNs are also an important target of the inner ear regeneration. For patients who do not have residual hearing, a cochlear implant (CI) has been the only solution to restore their hearing. As CIs function through direct stimulation of SGNs, restoration of hearing by CIs depends on the remaining SGNs. However, only a small fraction of remaining SGNs is required for CIs to perform [96]. The minimum required number of SGNs for CIs to effectively function is estimated as few as 3,500 [96, 97], whereas the number of SGNs in normal adult human is about 35,000 [98]. Therefore, the regeneration of even a small number of SGNs in a damaged ear has a significant clinical impact in relation to cochlear implants. Compared to the hair cells, the regeneration of SGNs seems realistic, because the transplantation of neural precursors derived from mouse [99] and human [91] ESCs has shown to improve the auditory brain stem responses.
In earlier trials, undifferentiated ESCs were transplanted with or without co-transplanted neural tissue [100–103], showing survival in the cochlea, partial differentiation into ectodermal/neural fate, and migration in the inner ear.
To be more specific to neural tissue regeneration, neural induction methods were adopted including simple monolayer culture [104, 105], stromal cell-derived inducing activity (SDIA) method (coculture with PA6 cells, a stromal cell line derived from skull bone marrow) [99, 106–109], or embryoid body formation followed by specific growth factors [91]. Okano et al. transplanted SDIA-induced neural progenitor cells derived from mouse ESCs into the modiolus of normal and deafened guinea pigs and showed the functional recovery by the improvement of ABR threshold [99]. Nishimura et al. first reported the transplantation of iPSC-derived neural progenitors into mouse cochlea, using SDIA as a neural-inducing method [108, 110]. Of note, a subset of transplants expressed vesicular glutamate transporter 1 (vGluT1), which marks glutamatergic neurons in the SGNs. Chen et al. showed functional recovery by the transplantation of human ESC-derived neural progenitor [91]. Human ESC-derived embryoid bodies were exposed to FGF3 and FGF10 and then replated and cultured on gelatin-coated dishes in the presence of bFGF and Sonic Hedgehog, followed by the supplementation of neurotrophin-3 (NT-3) and brain-derived neurotrophic factor (BDNF) to induce otic neural progenitors (ONPs). These ONPs were transplanted into the modiolus of auditory neuropathy model gerbils and gained partial improvement of ABRs.
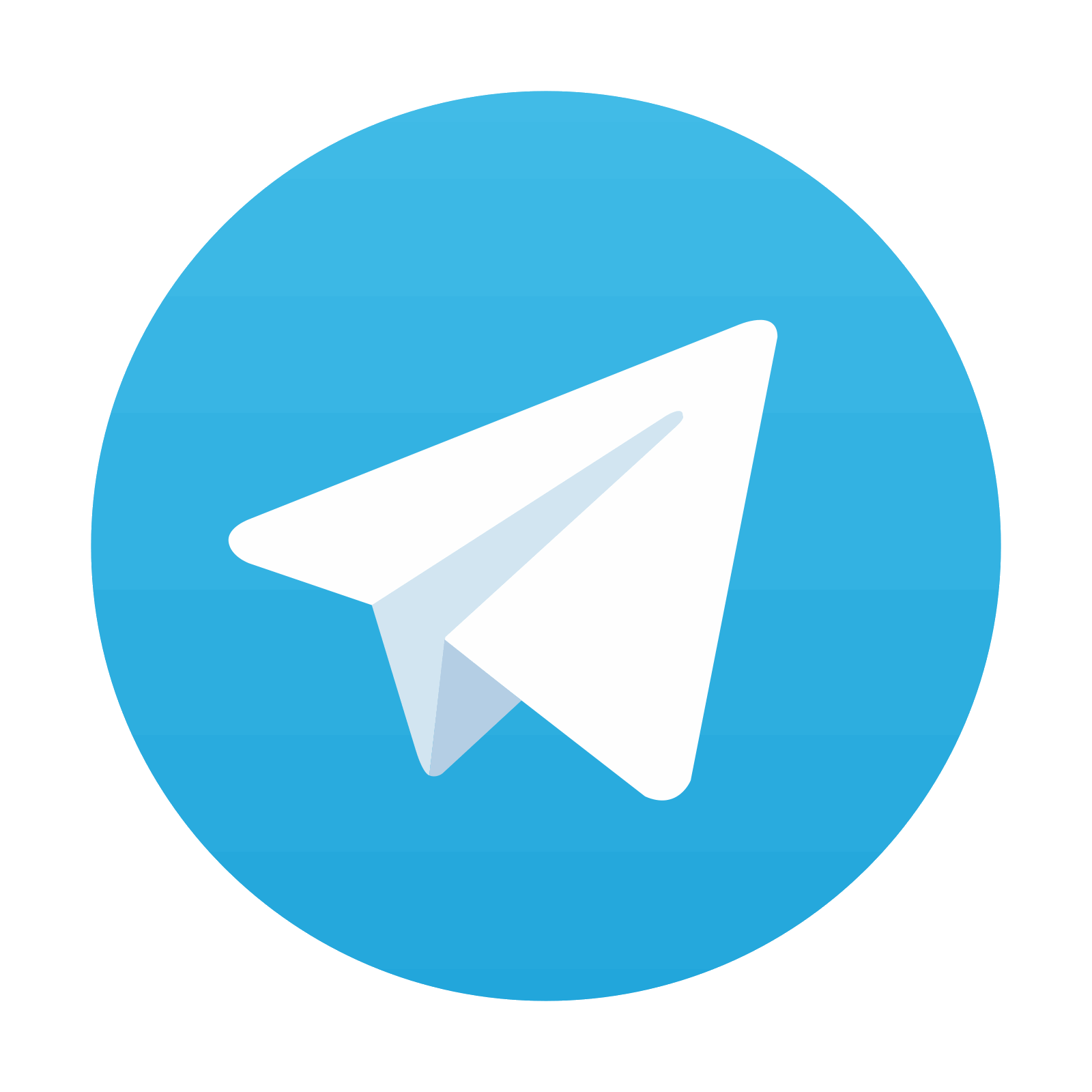
Stay updated, free articles. Join our Telegram channel
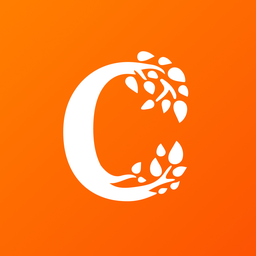
Full access? Get Clinical Tree
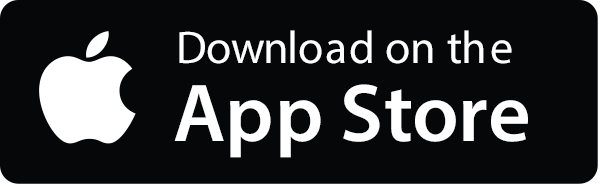
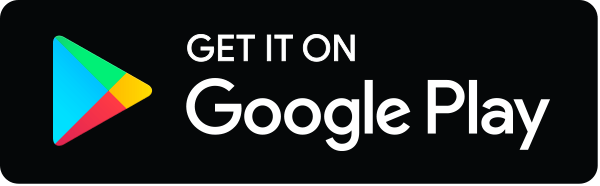