(1)
Charlotte Eye Ear Nose & Throat Associates, Charlotte, NC, USA
Abstract
The 4-aminoquinolines are weak bases that are completely absorbed from the gastrointestinal tract, sequestered in peripheral tissues, metabolized in the liver to pharmacologically active by-products, and excreted via the kidneys and the feces. The parent drugs and metabolites are excreted with a half-life of elimination of approximately 40 days. However, slow release from sequestered stores of the drugs means that after discontinuation, they continue to be released into the plasma for years. Correct dosing is based on the ideal body weight of the patient, which depends on height. The 4AQs diminish autoimmunity without compromising immunity to infections.
Abbreviations
4AQR
4-Aminoquinoline retinopathy
4AQs
4-Aminoquinolines (chloroquine and hydroxychloroquine)
ABW
Actual body weight
APC
Antigen presenting cell
BCVA
Best corrected visual acuity
C
Chloroquine
CpG ODN
CpG oligodeoxynucleotide
CV
Color vision
CYP
Cytochrome P450 enzymes
DFE
Dilated fundus examination
DNA
Deoxyribonucleic acid
ERK
Extracellular signal-regulated kinases
FP
Ferriprotoporphyrin IX
GVF
Goldmann visual fields
HC
Hydroxychloroquine
HIV
Human immunodeficiency virus
HMG-CoA
3-Hydroxy-3-methylglutaryl-coenzyme A reductase
IBW
Ideal body weight
IFN
Interferon
IL
Interleukin
LD50
Lethal dose 50
LDL
Low-density lipoprotein
M
Mole
mRNA
Mitochondrial ribonucleic acid
NG
Not given
RA
Rheumatoid arthritis
RNA
Ribonucleic acid
RPE
Retinal pigment epithelium
SARS
Severe acute respiratory syndrome
SLE
Systemic lupus erythematosus
TLR
Toll-like receptor
TNF
Tumor necrosis factor
TNF-α
Tumor necrosis factor α
V
Volume of distribution
This chapter covers the pharmacology of chloroquine and hydroxychloroquine, which is similar for both drugs [1], but the details are different. For example, both drugs are partially excreted in feces, but the proportions differ slightly—8–10 % for chloroquine and 15–24 % for hydroxychloroquine. Generally, whatever is said in this chapter about one drug can be assumed to apply to the other unless otherwise specified [1, 2]. Because both drugs are derivatives of a 4-aminoquinoline (4AQ) nucleus, they are referred to as 4AQs, and the retinopathy that they can cause is termed 4-aminoquinoline retinopathy (4AQR) [3]. Commonly used abbreviations in this chapter are collected in “Abbreviations” for reference. Each term will be first used in its full form, along with its abbreviation.
2.1 History
In the 1600s, the Jesuits who proselytized Chile discovered from the Incas that the bark of the cinchona tree can cure malaria [4, 5]. Additional medicinal qualities of cinchona bark were described in the 1700s, and the British and Dutch transplanted these trees to Javan plantations in the early 1900s for the production of quinine. In 1894, Payne described the use of quinine to treat systemic lupus erythematosus (SLE) [6]. Other alkaloids contained in cinchona bark, such as pamaquine, were also successfully used to treat SLE [5].
When the Japanese army occupied Java in World War II, the natural supply of quinine was lost, and synthesis of antimalarials was pursued in the United States [7]. Quinacrine, a 9-aminoacridine compound, was first used, but had the unpleasant side effect of staining the skin and sclera yellow in a manner indistinguishable from icterus [8–10]. The 4AQs, chloroquine and hydroxychloroquine, were found to be effective as antimalarials and did not discolor the skin. Chloroquine was first synthesized in 1934 by Andersag of I.G. Farbenindustrie in a German effort to find drugs better than quinine [11]. The Germans lost interest in the drug when they judged it to be too toxic for use in man, but the Americans restudied the drug and found it to be effective against malaria and sufficiently safe [3, 7, 12]. Hydroxychloroquine was synthesized in 1946 and proposed as a safer alternative to chloroquine in 1955 [13]. Resistance to chloroquine as an antimalarial became a problem in some parts of the world in the 1980s.
In World War II it was observed that servicemen with rashes and inflammatory arthritis who took quinacrine and chloroquine for malaria prophylaxis experienced improvement in their autoimmune conditions [14]. In 1951, Page used quinacrine to treat arthritis and autoimmune dermatologic conditions [15]. Later chloroquine and then hydroxychloroquine were also noted to favorably affect patients with rheumatologic diseases. Over time, both have been widely adopted for these uses. They are commonly used in patients with rheumatoid arthritis (RA), SLE, discoid lupus erythematosus, polymorphous light eruptions, solar urticaria, recurrent basal cell carcinoma of the skin, porphyrea cutane tarda, antiphospholipid antibody syndrome, and more than 20 other rarer conditions [11, 16–20].
The side effects other than retinopathy of the 4AQs are discussed in Chap. 3. Chapters 4 through 6 cover aspects of retinopathy. The 4AQs and quinacrine can cause retinopathy with the order of frequency chloroquine > hydroxychloroquine > > quinacrine. Chloroquine retinopathy was first described by Hobbs in 1959 [21]. Hydroxychloroquine retinopathy was first described by Braun-Vallon in 1963 [22, 23]. Quinacrine retinopathy is so rare that some have said that it does not exist [5, 24]. Nevertheless, it does, identical in its funduscopic appearance to 4AQR, and was described in 2004 [25].
2.2 Chemistry
The parent molecule for the antimalarials is quinine. Both chloroquine (C18H26ClN3) and hydroxychloroquine (C18H26ClN3O) are alkylated 4-aminoquinolines (4AQs) (Fig. 2.1) [6]. Chloroquine is 7-chloro-4(-4-diethylamino-1-methylbutylamino) quinoline and hydroxychloroquine is its hydroxyl derivative. Chloroquine and hydroxychloroquine have molecular weights of 320 and 336, respectively [26, 27]. Both chloroquine and hydroxychloroquine are amphiphilic weak bases based on two fused aromatic rings having conjugated double bonds, the 4-aminoquinoline nucleus (Fig. 2.1). Both drugs cross cell membranes well [16, 28, 29]. Hydroxychloroquine is more polar, less lipophilic, and has more difficulty diffusing across cell membranes [6, 30, 31]. The 4AQs lack the third benzene ring that is part of the acridine nucleus of quinacrine (Fig. 2.1) [6].


Fig. 2.1
Chloroquine and hydroxychloroquine are 4-aminoquinolines. Quinacrine has a side chain similar to that in chloroquine, but is based on an acridine nucleus
Useful Conversion Factors
In the literature on chloroquine and hydroxychloroquine, some articles express concentrations in μg/mL or ng/mL and others in M/L. The molecular weights of chloroquine and hydroxychloroquine are 320 and 336, respectively; so one mole of chloroquine weighs 320 g and one mole of hydroxychloroquine weighs 336 g. It follows that 1 × 10−6 M of chloroquine and hydroxychloroquine is equivalent to 320 ng/mL (0.320 μg/mL) and 336 ng/mL (0.320 μg/mL), respectively. Conversely, one μg/ml of chloroquine and hydroxychloroquine is equivalent to 3.125 × 10−6 and 2.97 × 10−6 M/L, respectively.
Hydroxychloroquine is more soluble than chloroquine, but both are water-soluble [32]. Chloroquine has two basic groups corresponding to the quinoline-ring nitrogen and the diethylamino side-chain nitrogen with ionization constants of 8.1 and 10.2, respectively [33–36]. At a physiologic pH of 7.4, 18 % of chloroquine is monoprotonated but still soluble in lipid and able to traverse cell membranes. However, biprotonated chloroquine, as occurs in a lysosome at a pH of 4–5, is sequestered and prevented from traversing back out to the cytoplasm (see Chap. 1) [34, 37]. Although the amount of free drug present in the plasma is miniscule at the physiologic pH, it is this form of the drug that determines the distribution of the drug between the plasma and the tissues [36]. Induction of acidosis increases the concentration of the drug in the plasma and erythrocytes but does not change drug concentration in the tissues, which have large reservoirs at steady state.
The chemical and brand names of the antimalarial drugs are listed in Table 2.1. Chloroquine diphosphate is the oral form of the drug, and chloroquine hydrochloride is the form used intramuscularly for malaria-induced coma [38]. Neither 4AQ is permitted for routine clinical use in Japan following multiple lawsuits over retinopathy in the 1970s [39]. Hydroxychloroquine is more commonly used in the United States [6]. Chloroquine is rarely used in the United States after multiple lawsuits in the 1960s led Winthrop Laboratories to withdraw the drug’s rheumatic indications, but continues to be commonly used in Canada, Mexico, Brazil, Europe, Poland, Turkey, South Africa, and Asia [1, 2, 6, 39–47].
Table 2.1
Chemical and brand names of antimalarial drugs
Chemical name | Brand name |
---|---|
Chloroquine phosphate | Aralen, Bemaphate, Chinamine, Delagil, Gontochin, Imagon, Iroquine, Klorokin, Luprochin, Resoquine, Sanoquin, Tanakan, Tresochin, Tochin |
Chloroquine diphosphate | Avloclor and Resochin |
Chloroquine sulfate | Amokin, Arechin Arthrochin, Artrichin Bemaco, Bemaphate, Bemasulph, Nivaquine, Resoquine |
Hydroxychloroquine sulfate | Axenal, Dolquine, Ercoquin, Plaquenil, Polirrheumin, Quensyl |
Quinacrine hydrochloride | Acriquine, Atabrine, Atebrin, Chinacrin, Erion, Itaichin, Mepacrine, Palacrin, Metoquine |
Amodiaquine dihydrochloride | CAM-AQ1, Camoquinal, Camoquine, Flavoquine, Miaquin, |
Quinine sulfate | Qualaquin |
Structure-activity studies of many derivatives of the 4AQs show that halogen substitutions at any position other than seven (Fig. 2.1) reduce pharmacologic activity and toxicity [12]. An aryl rather than an alkyl side chain decreases the therapeutic ratio [12]. Increasing alkyl side-chain length above five carbons decreases the therapeutic ratio and increases toxicity [12].
Chloroquine binds to nucleic acids by electrostatic forces, hydrogen bonds, and van der Waals forces [48]. As a cation at physiologic pH, it binds ionically to melanin, which is a polyanion with many negatively charged carboxyl groups and ortho-semiquinone groups [35, 49–52]. Other binding forces to melanin include van der Waal’s forces between the aromatic rings of chloroquine and the indole nuclei of melanin as well as charge transfer complexes in which melanin acts as an electron acceptor [35, 50–54]. The interactions of hydroxychloroquine and melanin parallel those of chloroquine.
Melanin and the 4-Aminoquinolines
Melanin is a name for a family of pigments which are polyanionic polymers formed from the oxidation of tyrosine in cellular vesicles called melanosomes [55]. The term is descriptive, not chemical. Eumelanin is brown or black. Pheomelanin is red or yellow and occurs in red hair [56]. Melanins occur in the retinal pigment epithelium (RPE) of the eye, in the inner ear, and in the substantia nigra of the brain. The highest concentrations are in the eye [55, 56]. The functions of melanin in the eye are to absorb light, preventing scatter, and to protect against free radicals [29, 56]. Although choroidal melanin seems to be synthesized throughout life, melanin in the RPE is synthesized for a brief interval of fetal and perinatal life with little production thereafter [56–58].
The interaction of melanin with 4AQs is complex. Some have written that increased melanin is related to toxicity [55]. Others have denied this or maintained an agnostic viewpoint pending further evidence [29, 54, 56]. Also, the possibility of a protective effect has been raised. The mechanism suggested is that melanin binds the drug and prevents formation of lamellar bodies until its binding capacity is exceeded [19, 51, 56, 59–62]. It has been hypothesized that the extensive binding of 4AQs to melanin produces high local gradients of the drugs that may have importance in identifying cell types that are particularly affected [63]. For example, calculations suggest that the effective concentration of chloroquine in the cytoplasm of the RPE may be in the range of 10−5 to 10−4 M/L [63]. Chloroquine accumulates in the uveal tract of pigmented animals, but not albino animals, but both types of animals develop 4AQR [64, 65].
Melanin content in the RPE decreases with age [58]. In the first two decades of life, an average of 8 % of cytoplasmic volume is occupied by melanin, but only 3.5 % after age 40 [66]. The decrease is thought to occur by the degradation of melanin, after damage by light or free radicals, into complex granules containing melanolysosomes and melanolypofuscin. The relationship of decreasing melanin and increasing risk of 4AQR with increasing age is intuitive but speculative.
The 4AQs have chiral carbons about which the side chains can be arranged so as to be nonsuperimposable mirror images of each other [67]. These stereoisomers, called enantiomers, are labeled as R(−) or S(+) forms. Clinically used 4AQs are racemic mixtures with equal amounts of R(−) and S(+) forms [67].
Both enantiomers of chloroquine are equipotent in vitro and in a duck model of malaria. In mice and rats S(+) chloroquine was more potent than R(−) chloroquine, possibly due to stereoselectivity in distribution of drug throughout the body [12, 37, 68, 69].
S(+) chloroquine is more highly bound to plasma proteins than R(−) chloroquine (67 % versus 35 %) [68]. Sequestration of the R(−) enantiomer in ocular tissues is greater than that of the S(+) enantiomer [67]. The toxicity of the S(+) enantiomer is greater than the R(−) enantiomer in mammals [69]. The S(+) enantiomer for both chloroquine and hydroxychloroquine is excreted by the kidneys preferentially compared to the R(−) enantiomer [55, 67, 68]. Because renal failure is associated with 4AQR, it is possible that S(+) chloroquine and S(+) hydroxychloroquine are more toxic in humans than R(−) chloroquine and R(−) hydroxychloroquine, respectively [55].
2.3 Pharmacokinetics and Tissue Distribution of the 4-Aminoquinolines
2.3.1 Absorption
Nearly complete absorption of 4AQs after an oral dose occurs within 2–4 h (Fig. 2.2) [2, 3, 12, 37, 70]. In fasting subjects the absorption of oral chloroquine was 89 ± 16 % and of hydroxychloroquine was 74 ± 13 % [70, 71]. Absorption is relatively unaffected by concomitant ingestion of food. However, intersubject variability of 30–100 % has been reported in extent of absorption, which may explain in part the individual variability of 4AQ effectiveness and toxicity [4, 71, 72].


Fig. 2.2
Chloroquine bioavailability and elimination in man. A single dose of chloroquine was given intravenously (blue circles), as an oral solution (yellow triangles), or as tablets (purple squares). Panel A shows plasma concentration of chloroquine and panel B chloroquine metabolite. The near superposition of the three curves in panel A is evidence of near-complete absorption of chloroquine from the gastrointestinal tract. Slow elimination is also indicated. Data from Gustafsson [70]
2.3.2 Distribution and Pharmacokinetics
The pharmacokinetics of chloroquine and hydroxychloroquine are similar [38, 73–75]. However, the explanation is complicated because of their differential sequestration in various tissues of the body and continuously evolving tests for detection of the drugs in plasma that are increasingly sensitive [37, 75, 76]. As a result, estimates of pharmacokinetic parameters have varied widely over the years [53, 75]. For example, the terminal half-life of chloroquine has been reported variously to be between one and 157 days [53, 70, 75, 76]. In addition, dose-dependent kinetics have been reported by some, while others have claimed that this interpretation arises from artifacts of insensitive detection methods [37, 75–77]. In the 1980s a two-compartment model was thought to best describe the pharmacokinetics of the 4AQs [2]. Now a model with three compartments is considered to be more accurate [37, 75].
The 4AQs have a large volume of distribution (V) due to extensive sequestration of the drugs by tissues. Plasma volumes of distribution up to 65,000 L for chloroquine and 44,257 L for hydroxychloroquine have been reported [71, 75, 77]. Normalized by body mass, reported volumes of distribution for chloroquine have ranged from 204 to 800 L/kg depending on the sensitivity of the method of detecting chloroquine [37]. Drug disposition proceeds in three phases—distribution from blood to tissues, equilibration between blood and tissues, and release from tissues back into blood [19]. These phases have half-lives of 3–8, 40–216 h, and 30–60 days, respectively [13, 37, 70, 75, 78].
The peak plasma concentration after an oral dose of chloroquine is 3–12 h [10, 32, 67]. Thirty-three to 70 % of the drug in plasma is protein-bound [2, 32, 38, 75, 79, 80]. The effects of hypoalbuminemia and altered immunoglobulin composition in patients taking 4AQs are not well understood [80].
The phase of terminal elimination has the greatest importance for the 4AQs as used in autoimmune diseases in which they are given for years and for which steady-state levels are the emphasis. The most commonly quoted median value for the terminal elimination half-life is 40 days [16, 37, 38, 75, 81]. The pharmacokinetics of chloroquine do not differ to a clinically important extent between black and white patients [11, 69, 79]. The average melanin content of a black person is estimated to be 1 g and for a white person is estimated to be 250 mg. This implies that melanin sequestration is not a large factor in systemic pharmacokinetics although the drug continues to elute from melanin at low levels for years after cessation of ingestion [49, 64, 71, 78, 82].
A lower daily dose of chloroquine and hydroxychloroquine leads to a lower plateau concentration in plasma (Fig. 2.3 and Table 2.2). Doses of chloroquine of 3.5–4.0 mg/kg/day based on ideal body weight (IBW) yield serum concentrations of 6 to 9 × 10−7 M/L. Doses of hydroxychloroquine of 6.0–6.5 mg/kg/day based on IBW yield serum concentrations of 1.4 to 1.5 × 10−6 M/L [2]. Daily dosing of chloroquine 250 mg/day produces plasma concentrations at equilibrium of 0.31 to 3.13 × 10−6 M/L depending on body mass [2, 38]. The dependence of time to reach equilibrium levels on daily dosing is schematically represented in Fig. 2.3. Because the half-life to reach a steady-state concentration is not the same for all daily doses, one may conclude that the mechanism of drug elimination is not a first-order process, but rather more complicated with some dependence of the elimination-rate constant on plasma-drug concentration (see Chap. 1) [83, p. 314, [84].


Fig. 2.3
Schematically depicted dependence of final equilibrium plasma concentration and equilibration time on daily dose of chloroquine. The dependence of the final equilibrium concentration on body mass is not shown. However, for a given daily dose the final equilibrium concentration will be lower for a higher body mass. Similar relationships apply to hydroxychloroquine dosing. Data from Mackenzie [2]
Table 2.2
Plateau concentrations of 4-aminoquinolines according to daily dosing
Drug/reference | Dosing | Equivalent daily dosing for a subject with IBW of 60 kg (mg/kg/day) | Plateau plasma concentration (M/L) | Plateau blood concentration (M/L) |
---|---|---|---|---|
C [80] | 250 mg/week | 0.60 | 10−8 | a5 × 10−8 |
C [80] | 500 mg/week | 1.19 | 10−7 | a5 × 10−7 |
C [80] | 250 mg/day | 4.16 | 10−6 | a5 × 10−6 |
C [80] | 500 mg/day | 8.33 | 10−5 | a5 × 10−5 |
C [69] | 310 mg/day | 5.17 | 3.9 × 10−7 | 1.95 × 10−6 |
HC [175] | 224 mg/day | 3.73 | b2.5 × 10−7 | 2.0 × 10−6 |
HC [71] | 155 mg/day | 2.58 | b5.88 × 10−7 | 2.94 × 10−6 |
HC [71] | 310 mg/day | 5.17 | b1.18 × 10−8 | 5.88 × 10−6 |
2.3.3 Steady-State Concentration of 4-Aminoquinolines in Various Organs
The concentration of 4AQs in the tissues of the body after ingestion varies. In pigmented rats, the order of concentration of chloroquine after a single dose from greatest to least is uvea > liver > lung > kidney > vitreous > heart > skin > hair > brain > blood > serum [53]. The results are similar in rabbits and humans [11, 82]. Drug binding to melanin explains the differences between pigmented and albino animals [11]. The order of concentration of hydroxychloroquine in various tissues of albino rats is similar, except that concentrations in the albinotic uvea fall to the approximate level of the heart. In pigmented mammals, the eye has the highest concentration due to binding by melanin [74]. Similar results were found after chronic oral administration of chloroquine to albino and pigmented rats (Figs. 2.4 and 2.5) [74].



Fig. 2.4
Relative concentration of hydroxychloroquine in various tissues in rats. Tissue levels of hydroxychloroquine in albino rats receiving 40 mg/kg/day of hydroxychloroquine orally by stomach tube, 6 days a week, for 3 months. Groups of four or six animals were sacrificed on days 30, 61, 91, 99, and 106. Results shown project back (mathematically, at intervals) to day 1, as indicated by broken lines. The single curve labeled “Eye (hooded)” refers to pigmented rather than albino rats. The melanin in pigmented tissues sequesters hydroxychloroquine. Data from McChesney EW [74]

Fig. 2.5
Concentration of chloroquine in ocular tissues of pigmented rats. Panel A—Mean concentration of radioactivity in iris/ciliary body (blue line, circles) and choroid (orange line, triangles) at 24 h after repeated oral administration of 14C-chloroquine at daily doses of 28 mg (0.054 mmol)kg/L under non-fasting conditions in pigmented rats for 84 days. Each value represents the mean ± standard deviation for three rats. Compare the concentrations in iris/ciliary body and choroid to those found in the retina and cornea in panel B. Panel B—Mean concentration of radioactivity in retina (orange line, triangles), cornea (purple line, squares), and vitreous (blue line, circles) after repeated oral administration of 14C-chloroquine at daily doses of 28 mg (0.054 mmol)kg/L under non-fasting conditions in pigmented rats for 84 days. The concentration of chloroquine in the choroid is approximately 12 times higher in the choroid than the retina after the 84th dose. Data from Tanaka [54]
Limited information from cases of accidental death in persons taking chloroquine and suicides by chloroquine ingestion indicates similar distributions of 4AQs across various tissues in humans [11]. Tissue uptake as a function of dosage is nonlinear. In rats, a threefold increase in dosing leads to a 20-fold increase in hydroxychloroquine deposition in liver and spleen [85].
A useful way to understand the differential distribution of 4AQs across various tissues is to consider ratios of concentration in tissues compared to plasma concentration (Table 2.3). The concentration of chloroquine in the liver, spleen, and adrenal gland is 6,000–80,000 times that in plasma, depending on the species chosen for study—whether the species is pigmented or albinotic—and the regimen of drug administration [10, 32, 37]. Within a single organ there are large differences in drug concentration in particular tissues. For example, chloroquine concentrations in the uvea of the rat are 9–32 times that of the retina, which in turn has concentrations six to nine times that of the vitreous [49, 54]. In another example, within skin the 4AQs are more concentrated in the epidermis than the corium with a concentration ratio of 5:1 to 15:1 [86]. The variation reflects sequestration of 4AQs by lysosomes and melanin [37]. Millimolar levels of 4AQs can be found in lysosomes, and melanin can bind a quantity of 4AQs up to 3 % of its weight [37, 87].
Table 2.3
Relative distribution of chloroquine among tissues
Model | Tissue | Tissue-plasma concentration ratio | Study |
---|---|---|---|
Human | Fat, tendon, bone | 0.1 | |
Albino rat, human | Erythrocytes | 1.9–4.0 | |
Albino rat | Whole blood | 3.7 | Berliner [3] |
Albino rat | Brain | 4–31 | |
Albino rat | Muscle | 4–41 | |
Human | Skin | 6–200 | |
Albino rat | Heart | 150 | Berliner [3] |
NG | Leukocytes | 100–300 | |
Albino rat | Kidney | 670 | Berliner [3] |
Albino rat | Lung | 640 | Berliner [3] |
Albino rat | Liver | 420 | Berliner [3] |
There are clinically unimportant differences in tissue distribution of chloroquine and hydroxychloroquine [78]. For an identical dose of hydroxychloroquine and chloroquine, tissue levels of chloroquine are 2.5 times those of hydroxychloroquine [74]. Tissue-plasma concentration ratios for chloroquine in various albinotic animals range from 28 to 93. For hydroxychloroquine the analogous tissue-plasma concentration ratios range from 33 to 79. In contrast, for both drugs in pigmented animals the tissue-plasma concentration ratios are approximately 1,000–3,000 [11]. The 4AQs remain in human tissues for years. Five years after last ingesting chloroquine, chloroquine and its metabolite have been measured in the urine [85, 88].
2.3.4 Metabolism
Metabolism of 4AQs is by dealkylation in the liver (Fig. 2.6) [68]. To determine metabolites of chloroquine, carbon-14-labeled chloroquine was administered to monkeys, after which 12 labeled metabolites were detected. The two most important were desethyl chloroquine and bisdesethyl chloroquine, both of which have pharmacologic activity and are thought to be approximately as toxic as the parent compounds [11]. The differential efficacy and toxicity of the metabolites of 4AQs has not been studied, but a suggestion has been made that desethylhydroxychloroquine might have a higher therapeutic ratio than the parent compound hydroxychloroquine [77].


Fig. 2.6
Metabolism of chloroquine by dealkylation. Both metabolites are found in plasma and urine and both have activity similar to the parent compound. Data from Projean [89]
The quinoline ring is resistant to degradation by cytochrome P450 enzymes (CYP) CYP2C8 and CYP3A4, which mediate 80 % of the total metabolism of 4AQs [38, 89–91]. The metabolism of chloroquine and hydroxychloroquine differs only in the number of metabolites produced (Fig. 2.7) [92]. Thirty percent to 79 % of an oral dose of a 4AQ is metabolized and 21–70 % is excreted without metabolism [24, 69, 91, 93].


Fig. 2.7
Chemical structures of the three major metabolites of chloroquine and hydroxychloroquine. Chloroquine has two metabolites, whereas hydroxychloroquine has three
Desethylchloroquine concentration after a dose of chloroquine reaches 40–48 % of chloroquine concentration. Bisdesethylchloroquine concentration reaches 10–13 % of chloroquine concentration [38, 68, 93, 94]. At steady state the ratio of chloroquine to desethylchloroquine was 7.2 ± 1.88 while the ratio of hydroxychloroquine to desethylhydroxychloroquine was 1.75 ± .37 [77].
Inhibition of cytochrome P450 isoenzymes by other drugs and variation across individuals in expression of the isoforms may explain the variation in levels of the two metabolites after ingestion of the same dose of chloroquine or hydroxychloroquine [77, 89]. For example, ketoconazole, an inhibitor of CYP3A4, inhibited the formation of desethylchloroquine by 33 % and 45 % at concentrations of 1 × 10−6 and 1 × 10−5 M/L, respectively [93]. Cimetidine, another CYP3A4 inhibitor, increased the half-life of an oral dose of chloroquine by 48 % [95]. The elimination half-lives of chloroquine, desethylchloroquine, and bisdesethylchloroquine are all from 20 to 60 days. The half-lives of elimination of the desethyl metabolites of the 4AQs are longer than those of the parent compounds. [77] Chloroquine and metabolites can be found in urine for months after a single oral dose [68].
Caution should be exercised in considering the results of testing in animal models. The results may not translate directly to human metabolism. For example, the dog metabolizes chloroquine by glucuronidation in a manner unlike some other species that leads to a relatively short half-life [11].
2.3.5 Excretion and Storage
4AQs are excreted by the kidney and the liver [96]. For both chloroquine and hydroxychloroquine, approximately 40–60 % is excreted as unchanged or metabolized drug through the kidneys, 8–25 % is excreted in unchanged or changed form in the feces, 5 % is sloughed off through the skin, and 25–45 % is stored long term in lean body tissues (Table 2.4) [3, 11, 37, 38, 53, 73, 74, 80]. Kidney or liver dysfunction decreases excretion of 4AQs and leads to greater drug retention and higher risk of retinopathy [80]. In the anuric patient, compared to subject with normal renal function, the equilibrium level of chloroquine is 70 % higher and for hydroxychloroquine is 25–30 % higher [71]. Declining renal function may be one mechanism by which age becomes a risk factor for retinopathy.
Table 2.4
Excretion of 4-aminoquinolines
Fate | Chloroquine (%) | Hydroxychloroquine (%) | References |
---|---|---|---|
Excreted unchanged in urine | Range 10–60; median 38 | Range 6–60; median 23 | |
Excreted as metabolites in urine | Range 7–31; median 18 | 17 | |
Excreted in feces | 8–10 | 24–25 | |
Sloughed off in skin | 5 | 5 | Mackenzie [80] |
Stored long term in lean tissues | 45 | 45 | Mackenzie [80] |
Alkalinization of urine decreases excretion [32]. Acidification of the urine by oral ingestion of ammonium chloride can increase renal excretion 20–80 % [85]. Intramuscular injection of dimercaprol can also increase urinary excretion of chloroquine [85]. Neither of these approaches has been adopted in clinical practice as practical methods to treat chloroquine retinopathy.
2.4 Clinical Uses and Dosing
The 4AQs are commonly used in rheumatologic conditions because they are effective in a high proportion of cases and have fewer side effects than gold, azathioprine, penicillamine, or levamisole [97–100]. The diagnoses for which 4AQs are most prescribed are rheumatoid arthritis (RA) and SLE, which together account for 82–95 % of the cases [23, 101, 102]. An epidemiologic survey, found that 60.1 % of patients with RA and 92.2 % of patients with SLE take hydroxychloroquine at some point in the course of their disease [61].
Antimalarials have a different mechanism of action than other immunomodulating therapies making them useful in combination therapy [42, 103–105]. In RA and SLE it is commonly observed that 60–95 % of patients taking chloroquine have a clinical response measured by subjective improvement, objective responses in laboratory measurements, activities of daily living, and reductions in flares of disease [8, 10, 37, 71, 106–112]. Although initially embraced for patients with milder forms of SLE and RA [10], the recent trend is that 4AQs are being used in a higher proportion of patients with SLE; some have advocated their use in all SLE patients [24, 104]. Many reports pool all patients taking 4AQs together under the assumption that the disease for which the drug is taken is immaterial to the risk of retinopathy [62, 113, 114].
Clinical efficacy may be greater in SLE than in RA [115]. In 15 % of patients the clinical response is dramatic [37]. The erythrocyte sedimentation rate, C-reactive protein, and serum IgG levels typically decrease in patients with autoimmune diseases treated with 4AQs. Occasionally, long-term remission of autoimmune disease is possible with no progression of radiologically documented joint disease [116]. In SLE, use of 4AQs has also improved survival [117]. This may be due to the antiatherogenic and antithrombotic effects of 4AQs as well as their beneficial effects on lipid profile and osteoporosis [103, 104]. When the drugs are stopped, beneficial effects last for several weeks to months, but disease activity generally recurs [116]. There is typically no increase in rates of opportunistic infection. Chloroquine prevents an immunologic response to antigenic proteins requiring digestion, but not the response of T cells to predigested antigenic peptides [118].
Full effects of the 4AQs may take 3–6 months to develop [37, 38, 106, 107, 116, 118–120]. Some have attributed this to a pharmacodynamic mechanism involving an immune process that requires the observed time to develop. However, the effect is predictable based on pharmacokinetics and the time required to saturate lysosomes (see Chap. 1) [83, 94, 121, 122]. The delayed onset of therapeutic effect of the 4AQs after initiation of therapy can be shortened by using a higher loading dose in the first weeks to months at the price of an increased frequency of gastrointestinal side effects [10, 38, 119, 121, 123]. A doubling of the daily dose is common in the first part of a course of rheumatologic therapy [124]. Beginning with the maintenance dose leads to a slower onset of action [121, 123].
Some clinicians give patients a drug holiday during summer as a way of assessing if the drug is still required for control of disease activity [1, 123]. This practice should also reduce the probability of developing 4AQR. Rheumatologists frequently decrease the dosing of 4AQs over time. In one series of 758 patients, 84 % of patients begun on 400 mg/day of hydroxychloroquine had their daily doses reduced over time. This is important, since the most common intervention by ophthalmologists is to suggest dosage reductions, not cessation of 4AQs, in response to concerns of retinopathy [125–127].
Approximately 20–50 % of patients with SLE and 75 % of patients with RA eventually have cessation of the drug by 6–10 years of follow-up, mainly because of inefficacy in the case of RA and because of disease remission in the case of SLE [97, 115, 125, 128, 129]. The rate of discontinuation of 4AQs is highest in the first 2 years. In the case of hydroxychloroquine, by 2 years approximately 34 % of patients with SLE and 54 % of patient with RA will have discontinued the drug [8, 125, 128]. Discontinuation of hydroxychloroquine may be less common in patients treated for SLE than RA. In one series, the overall 5-, 10-, and 15-year discontinuation rates were 20 %, 38 %, and 58 %, respectively [115]. Approximately 5 % of patients stop treatment because of ocular problems such as blurred vision or corneal deposits and 5 % stop because of gastrointestinal complaints, rashes, and other side effects. Rates of stopping 4AQs are dependent on the physician, the patient, and their relationship [97]. Some physicians and patients are more willing than others to tolerate less-than-complete control of disease activity and some degree of side effects [97].
Chloroquine is commonly given in doses of 100–250 mg/day for prophylaxis against malaria [130]. It is given as 1,500 mg over 3 days as a treatment for an acute episode of malaria [131]. The most common doses of chloroquine and hydroxychloroquine in clinical use are 250 and 400 mg/day, respectively, regardless of height or weight of the patient [19, 24, 45, 97, 100, 126]. That is, cumulative doses greater than 100 times that used for prophylaxis or treatment of malaria are administered to patients with autoimmune diseases [11, 100, 115, 130]. Before 1980, dosing of 4AQs was excessive and largely responsible for the greater prevalence of 4AQR reported in this era [10]. For example, in 1978 it was recommended that 400 mg/day of hydroxychloroquine and 250 mg/day of chloroquine were acceptable doses for all adults weighing over 100 lb [10]. The threshold for IBW at which these doses are now recognized to be unacceptable is now recognized to be 135 lb, not 100 lb [32, 127, 132]. More sophisticated regimens seek dosing not greater than 6 mg/kg/day based on actual body weight (ABW) [97] or 6.0–6.5 mg/kg/day based on IBW for hydroxychloroquine [78, 133]. The analogous ceiling for chloroquine is 3.5 mg/kg/day based on IBW. The preferred dosing of 4AQs is based on IBW [80, 114]. If a patient’s ABW is less than the IBW based on height, then the ABW should be used to determine dosing [126].
A 250 mg dose of chloroquine phosphate contains 150 mg of chloroquine base [19, 24]. A 200 mg dose of hydroxychloroquine sulfate contains 155 mg of hydroxychloroquine base [1, 19, 24]. The relative potency and toxicity of the two 4AQs is inconsistently portrayed. Some have written that chloroquine is more potent and more toxic than hydroxychloroquine [20, 24, 134], but others have said the reverse. For example, 400 mg of hydroxychloroquine has been assumed to be equivalent to 500 mg of chloroquine [1, 134, 135], yet another report assumes that 400 mg of chloroquine is equivalent to 500 mg of hydroxychloroquine [134]. Other reports state that 400 mg of hydroxychloroquine is equivalent to 250 mg of chloroquine [10, 136].
The best analysis based on multiple forms of administration in different animal models and in human trials is that chloroquine and hydroxychloroquine are equipotent and that chloroquine is approximately twice as toxic [11]. That is, in acute toxicity experiments in animal models, the dose at which 50 % die (LD50) is approximately twice as high with hydroxychloroquine as with chloroquine (see Chap. 3) [11]. Likewise, in subacute and chronic toxicity studies, the tolerated dose for 50 % of animals is approximately twice as high with hydroxychloroquine as with chloroquine [11]. Although less rigorous, clinical series in humans suggests the same with a smaller percentage of side effects with hydroxychloroquine compared to chloroquine when the two are given in comparable dosages [137, 138]. Therefore, at the most commonly prescribed daily doses, chloroquine and hydroxychloroquine have been considered equally effective in treating autoimmune diseases with the therapeutic ratio favoring hydroxychloroquine (see Chap. 1).
Reports are inconsistent in the way they report drug concentrations. Plasma, serum, and whole blood concentrations have been used. They are not comparable; plasma concentrations are the lowest, and whole blood concentrations are the greatest. Serum concentrations exceed plasma concentrations because platelets concentrate 4AQs, and upon coagulation of the blood the platelet contents are released into the serum [139]. Plasma levels of chloroquine effective against malaria are 10−8 to 10−7 M/L [37, 140]. For rheumatological disease higher plasma levels are required—approximately 10−6 M/L [34, 37, 71, 89, 140, 141]. Serum concentrations of chloroquine in patients taking chloroquine 250 mg/day for long periods are in the range 6.25 × 10−7 to 1.25 × 10−6 M/L [142]. Therapeutic whole blood concentrations of HC for autoimmune diseases are 8 × 10−7 to 5 × 10−6 M/L, approximately five times higher than plasma concentrations [16, 37, 78, 89]. Using whole blood concentrations is not optimal because 4AQs are preferentially sequestered in erythrocytes and leukocytes which implies that hematocrit and white blood cell count will affect the whole blood concentration in potentially confounding ways. On the other hand, the precision of measurements is higher with whole blood than with plasma, possibly due to the technical difficulty of separating blood cells and platelets from plasma, making whole blood more suited for pharmacokinetic analyses [77].
In a study of patients with rheumatoid arthritis, patients with less morning stiffness and no rheumatoid factor had significantly higher blood concentrations of hydroxychloroquine than patients with more morning stiffness and presence of rheumatoid factor [143]. Other studies have not found a correlation between plasma concentration and clinical response [77, 139, 144]. In patients taking hydroxychloroquine for RA and SLE, daily dosing at 400 mg/day was associated with wide variability in whole blood concentrations [145]. In a study of 143 patients with SLE, those patients with inactive disease had average whole blood concentrations of 3.37 × 10−6 ± 1.64 × 10−6 M/L compared to 2.17 × 10−6 ± 1.40 × 10−6 in those patients with active disease [145]. Higher rates of efficacy can be assured if dosing is calibrated to yield a whole blood concentration of approximately 3.1 × 10−6 M/L [38, 145]. Variability may be due to lack of adherence to therapy or differences in absorption or metabolism of the drugs by individuals. Because of interindividual variability, in certain patients it may not be possible to reach therapeutic concentrations of 4AQs without exceeding daily dose thresholds based on IBW that are considered high risk for causing retinopathy [145]. Patients taking hydroxychloroquine for graft-versus-host disease have higher whole blood concentration targets of 5 × 10−6 to 1.5 × 10−5 M/L. Concerns over retinopathy in these patients are balanced against the more serious nature of their systemic disease. Effective plasma concentrations and effective in vitro concentrations of the drugs are not necessarily closely related, as the drug concentrations may be higher locally due to intracellular organelle sequestration [139, 140]. Other potential confounders include receptor concentrations, the influence of alcohol and smoking, genetic and gender influences, and age [77]. Perhaps because of inconsistency of results, few clinicians measure plasma concentrations of 4AQs to judge adequacy of a trial of drug [24, 139].
One therapeutic strategy used to reduce the risk of retinopathy yet obtain the beneficial disease modifying effects of antimalarial therapy is to combine chloroquine or hydroxychloroquine with quinacrine, which is not a 4-aminoquinoline and is considered to have a lesser risk of causing retinopathy. In practical terms, the patient is begun on hydroxychloroquine 400 mg/day or 6.5 mg/kg/day based on IBW, whichever is lower. After 2 months, if the autoimmune disease has not sufficiently responded, quainacrine 100 mg/day is added. After one additional month if a response has been achieved then the hydroxychloroquine is reduced to 200 mg/day [38]. Although the risk of retinopathy with quinacrine is less than with 4AQs, retinopathy has been associated infrequently with quinacrine [25].
2.5 Pregnancy and Lactation
Antimalarials cross the placenta and can concentrate in the eyes of fetal animals in mice and monkey models [64, 146]. Cord blood concentrations of antimalarials are similar to maternal blood levels [147, 148]. Toxicity in the fetus has not been reported. However, the number of mothers who have taken one of the drugs on a daily basis while pregnant and whose offspring have subsequently been examined for retinopathy has been small and the methods for checking retinopathy insensitive (Table 2.5) [149–151]. A randomized control trial with greater than 400 pregnancies per arm would be required to detect a difference in 5 % in pregnancy loss with alpha error of 0.05 and beta error of 0.8 [152]. Such a study is unlikely to be done, and therefore practice is likely to be guided by evidence of lesser quality. In case–control studies and prospective case series, the rates of spontaneous abortion, fetal death, fetal distress, and congenital malformations have not differed, although the studies have been small with little power to detect small differences in rates [152–154]. Flares of lupus among women who stop 4AQs during pregnancy are more frequent than in women who continue these drugs throughout pregnancy [152, 153]. The general consensus is that mothers using antimalarial drugs during pregnancy need not stop them as they do not appear to affect fetal health [147, 150, 153–156]. Dissenting opinion has been published, but it is based on anecdotal associations of fetal abnormalities in mothers taking 4AQs during pregnancy [8, 32, 71, 157].
Table 2.5
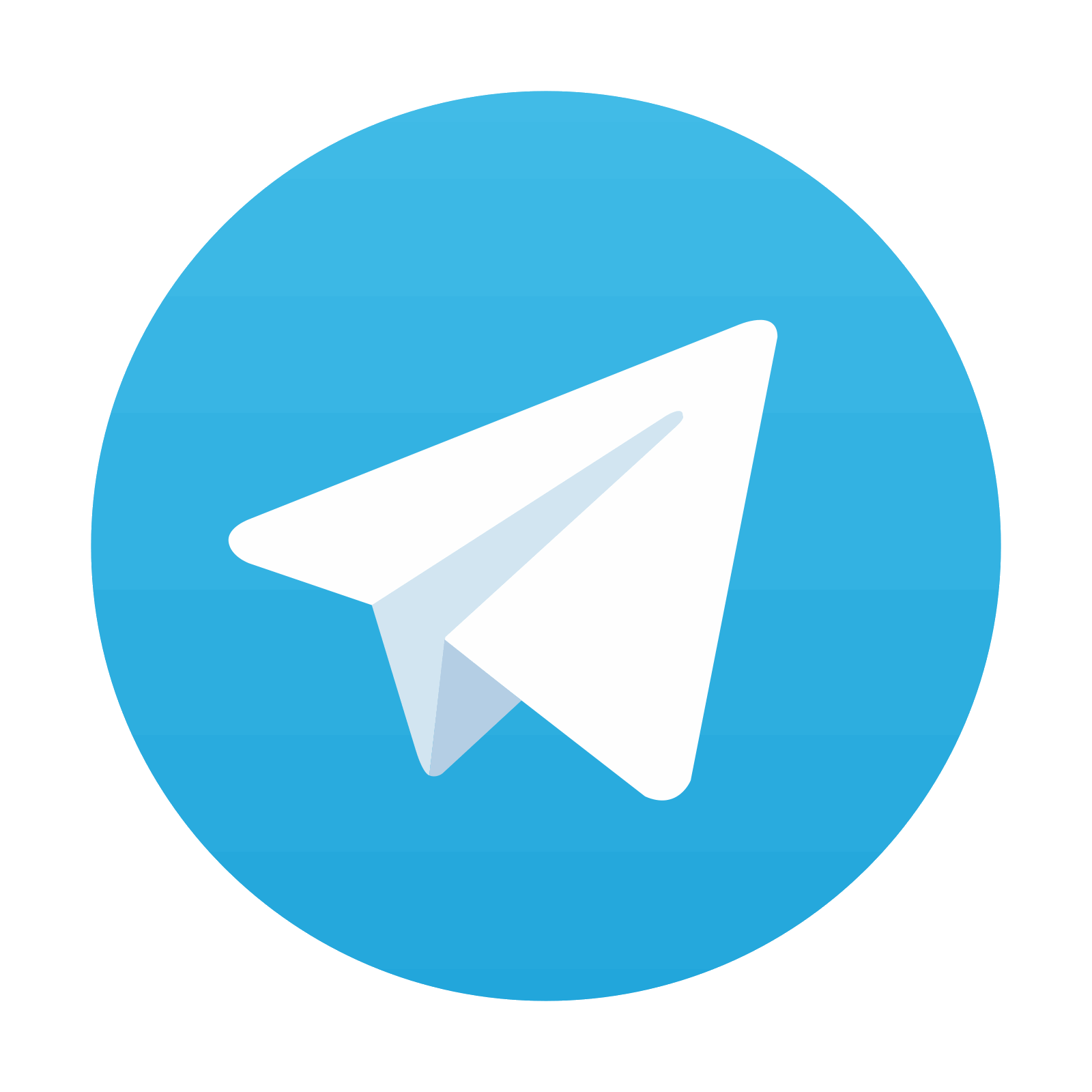
4-Aminoquinoline use in pregnancy and the risk of retinopathy in offspring
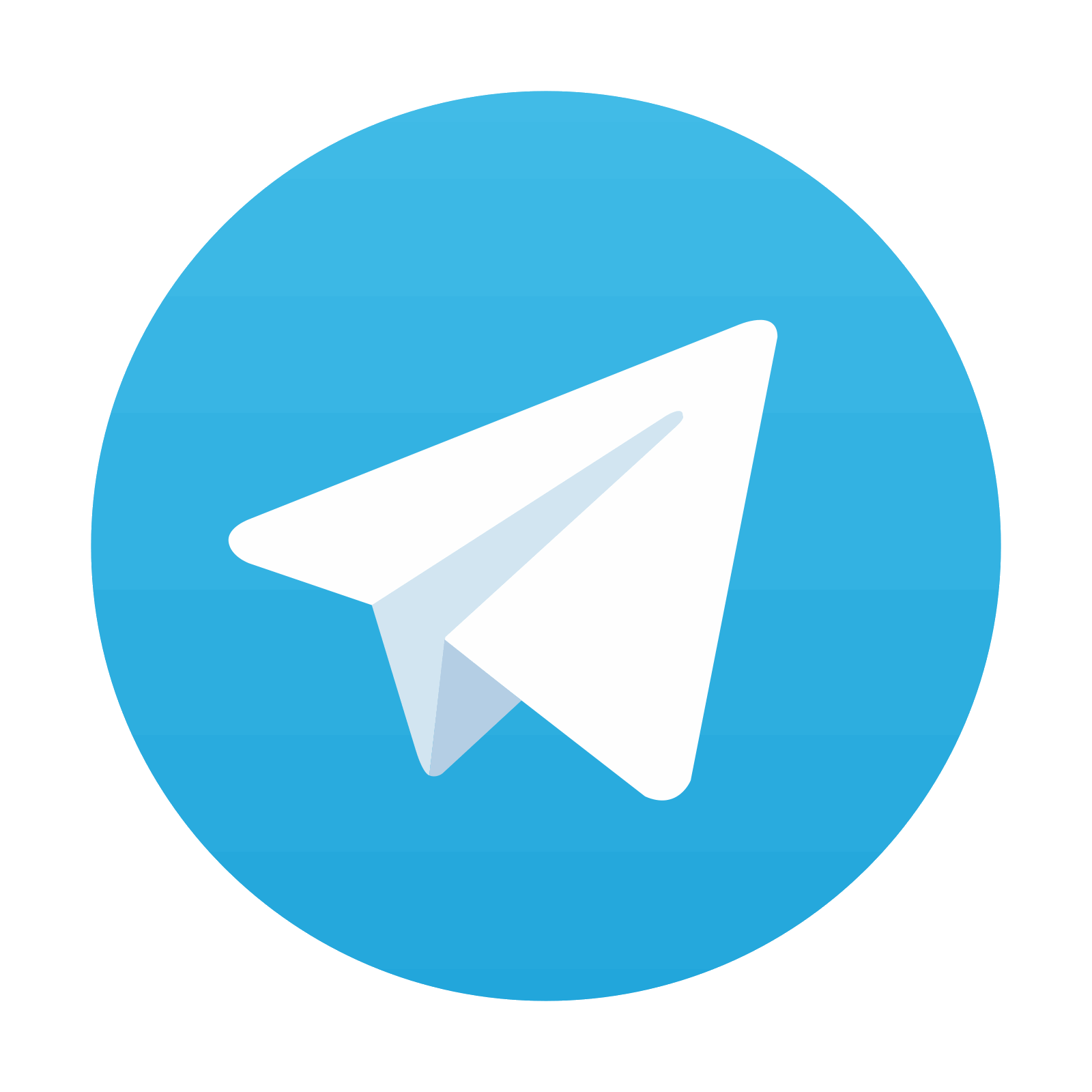
Stay updated, free articles. Join our Telegram channel
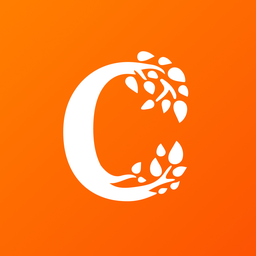
Full access? Get Clinical Tree
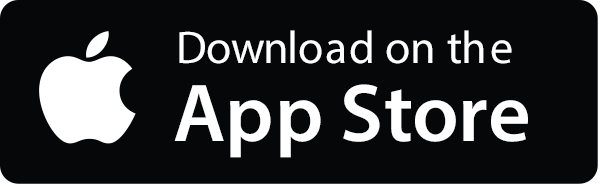
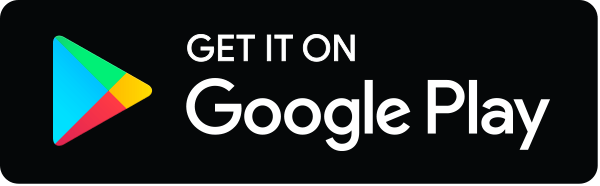
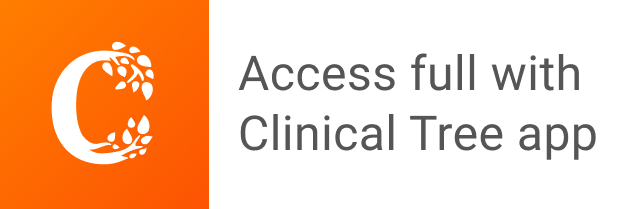