Obesity
High body mass index. Central or visceral obesity is quite important. Predisposing factors: abdominal circumference >94 cm in men and >80 cm in women; neck circumference >40 cm
Gender
Prevalence is higher in men than in women. Women present greater genioglossus muscle tone, which can be considered a defense mechanism designed to maintain upper airway permeability
Hormones
Estrogen and progesterone promote the maintenance of upper airway permeability (by improving muscle tone) as well as increasing respiratory drive. The androgens induce greater fat deposition and relaxation of the pharyngeal dilator muscles. Menopause also increases the risk of OSA
Age
The activity of the upper airway musculature is decreased with aging
Anatomical factors
Micrognathia and hypoplasia of the mandible are associated with the posterior positioning of the base of the tongue and with upper airway narrowing. Thickening of the lateral pharyngeal walls also causes upper airway narrowing
Genetic factors
Some risk factors, such as craniofacial structure, distribution of body fat, neural control of the upper airways, and central respiratory command, can be inherited
Posture and gravity
The dorsal decubitus position promotes the posterior positioning of the tongue and soft palate, thereby reducing the area of the oropharynx
Other causes
Acromegaly, Down’s syndrome, hypothyroidism, genetic syndromes, and deposition diseases (amyloidosis and mucopolysaccharidosis) can promote the narrowing of the upper airways, which are predisposing factors for OSA
In the presence of an anatomically compromised, collapsible airway, the sleep-induced loss of compensatory tonic input to the upper airway dilator muscle motor neurons leads to collapse of the pharyngeal airway. In turn, the ability of the sleeping subject to compensate for this airway obstruction will determine the degree of cycling of these events. Several of the classic neurotransmitters and a growing list of neuromodulators have now been identified that contribute to neurochemical regulation of pharyngeal motor neuron activity and airway patency.
Knowledge and understanding of the pathogenic basis, clinical presentation, and diagnosis of OSA are essential for the development of preventive, screening, and therapeutic strategies to reduce the public health burden of the disorder (Adams et al. 2001).
24.3 Influences of Wakefulness on Ventilatory Control
Remarkably, sleep apnea patients experience little or no problems with their breathing or airway patency while awake. In fact, the great majority of people with sleep apnea possess ventilatory control systems that are capable of precise regulation of their alveolar ventilation and arterial blood gases with extremely small variations from the norm throughout the waking hours. In addition, these healthy control systems, while awake, possess sufficiently sensitive feedback and feed forward controls to ensure precise coordination of chest wall and upper airway “respiratory” muscle recruitment so as to provide maximum airway diameter, low airway resistance, and optimum lung volumes and respiratory muscle lengths, regardless of the ventilatory requirement.
To underscore the importance of the “waking stimuli” to breath and to upper airway patency and to ventilatory control, consider the following qualitative influences of sleep on the control of breathing. Electrical activity from medullary inspiratory neurons, EMG activity of diaphragm and abductor muscles of the upper airway in healthy humans and/or in cats, shows reductions in amplitude upon the transition from awake to NREM sleep, usually accompanied by a mild-to-moderate hypoventilation (2–8 mmHg PaCO2) and two- to fivefold increases in upper airway resistance (Dempsey et al. 2002). Sleep induces consistently greater proportional reductions in the EMG activity in the upper airway versus chest wall pump muscles (Orem et al. 2002).
PaCO2 can be lowered substantially (using mechanical ventilation) during wakefulness with little or no disruption of breathing pattern; however, in NREM sleep, very small transient reductions in PaCO2 (even only to the waking level) result in significant apnea (Meza et al. 1998).
Sleep-disordered breathing leading to repeated bouts of ventilatory overshoots and undershoots and accompanying swings in arterial blood gases and intrathoracic pressure takes on many forms. Commonly, sleep-disordered breathing is divided into so-called “central” events, denoting an absence or marked reduction in central respiratory motor output to respiratory pump muscles, or “obstructive” events, which are comprised of respiratory efforts against a closed upper airway. However, as we discuss below, most cyclical sleep-disordered breathing events are driven by anomalies in both anatomical and neurochemical control of upper airway and/or chest wall respiratory musculature.
In short, the process is initiated because the wakeful state provides compensatory neuronal activation of dilator muscles in an anatomically compromised collapsible pharynx; accordingly, when this activation is lost at sleep onset, the airway narrows and/or collapses. However, the tendency to result (or not to result) in repeated cyclical apneas is the end product of multiple compensatory processes that vary markedly among and within individuals. Concepts have continued to evolve as we learn more about the neurophysiological mechanisms governing control of respiratory rhythm and its coupling with upper airway control and states of consciousness and applying these principles to human patients during sleep.
We will discuss the pathophysiology of OSA in three steps. First, we detail the varied structural and functional determinants of an anatomical predisposition for airway closure, an absolutely essential component for OSA. The second essential component is sleep. This section emphasizes the effects of the sleeping state on mechanisms underlying both obstructive and central apnea and ventilatory instability. Finally, we attempt to integrate anatomical deficits with mechanisms underlying central neurochemical control of breathing stability and compensatory neuromuscular control of upper airway caliber, to explain the repetitive nature of OSA.
24.3.1 Anatomical Determinants of Upper Airway in OSA
The pharyngeal airway is a complex structure that serves several purposes including speech, swallowing, and respiration. The human pharynx is composed of more than 20 muscles and divided into four sections that include the nasopharynx (from the nasal turbinates to the start of the soft palate), velopharynx (from the start of the soft palate to the tip of the uvula), oropharynx (from the tip of the uvula to the tip of the epiglottis), and hypopharynx (from the tip of the epiglottis to the level of the vocal cords) (Fig. 24.1). The human pharynx can be considered as a collapsible tube that is uniquely susceptible to collapse due to the presence of a floating hyoid bone, a longer airway, and a less direct route for inspired air to travel when compared to other mammals. The presence of soft tissues and bony structures, which increase extraluminal tissue pressures surrounding the upper airway, can predispose the pharynx to collapse. In contrast, the actions of pharyngeal dilator muscles maintain pharyngeal patency due to reflex pathways from the central nervous system and within the pharynx. The presence of these opposing forces suggests that increased pharyngeal collapsibility is due to alterations in anatomically imposed mechanical loads and/or in dynamic neuromuscular responses to upper airway obstruction during sleep.
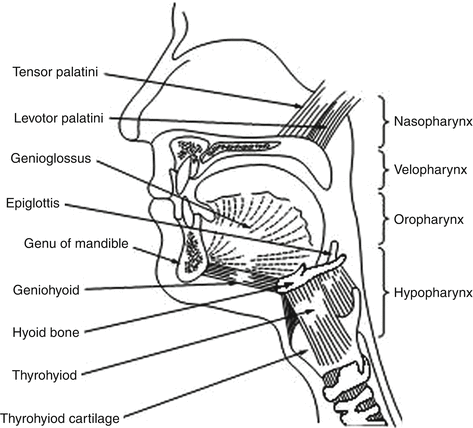
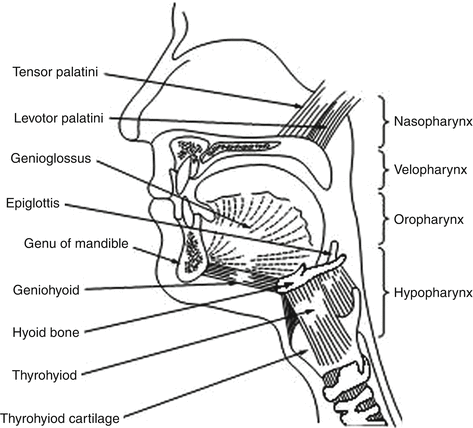
Fig. 24.1
Anatomy of the upper airway and the important muscles controlling the airway patency
24.3.1.1 Unique Anatomy of the Human Airway
The upper airway is a complex structure required to perform deglutition, vocalization, and respiration. In the human, this structure must also perform tightly controlled and complex motor behaviors required for speech. Upper airway obstruction in sleep is most prevalent in the human in part because the hyoid bone, a key anchoring site for pharyngeal dilator muscles, is not rigidly attached to skeletal structures (Friberg et al. 1997). In other mammals, the hyoid bone is attached to the styloid processes of the skull. Thus, the human pharynx has no rigid support except at its extreme upper and lower ends where it is anchored to bone (upper) and cartilage (larynx); therefore, pharyngeal cross-sectional area will vary with lumen pressure. Humans depend critically on the coordinated actions and interactions of over 20 skeletal muscles that dilate and stent open the oropharynx.
Beyond the hyoid arch, it is also pointed to the anatomical changes in the adult human upper airway during the evolutionary development of speech as a potential major contributor to OSA (Moser and Rajagopal 1987). Specifically, the gradual descent of the larynx to a position greatly inferior to the oropharynx separated the soft palate from the epiglottis in which the tongue encroaches significantly on the available space.
24.3.1.2 Sites of Airway Collapse
Studies using nasal pharyngoscopy, computer tomography and magnetic resonance imaging, or pharyngeal pressure monitoring have shown that one or more sites within the oral pharyngeal region are usually where closure occurs in most subjects with OSA, and this region is also smaller in OSA patients versus controls even during wakefulness (Schwab et al. 2005). Although the retropalatal region of the oropharynx is the most common site of collapse, airway narrowing is a dynamic process, varying markedly among and within subjects and often includes the retroglossal and hypopharyngeal areas (Tankersley et al. 1999).
24.3.1.3 Soft Tissue and Bony Structure Abnormalities
The recent use of quantitative imaging techniques has allowed advances that reveal important differences in both craniofacial and upper airway soft tissue structures in the OSA patient. The reduced size of cranial bony structures in the OSA patient includes a reduced mandibular body length, inferior positioned hyoid bone, and retroposition of the maxilla, all of which compromise the pharyngeal airspace (Bacon et al. 1990). Airway length, from the top of the hard palate to the base of the epiglottis, is also increased in OSA patients, perhaps reflecting the increased proportion of collapsible airway exposed to collapsing pressures (Malhotra et al. 2001). As expected, these craniofacial dimensions are primarily inherited, as the relatives of OSA patients demonstrated retroposed and short mandibles and inferiorly placed hyoid bones, longer soft palates, wider uvulas, and higher narrower hard palates than matched controls (Schwab et al. 1997).
Enlargement of soft tissue structures both within and surrounding the airway contributes significantly to pharyngeal airway narrowing in most cases of OSA. An enlarged soft palate and tongue would encroach on airway diameter in the anterior-posterior plane, while the thickened pharyngeal walls would encroach in the lateral plane. Volumetric time overlapped magnetic resonance imaging (MRI) or computer tomography (CT) images strongly implicate the thickness of the lateral pharyngeal walls as a major site of airway compromise, as the airway is narrowed primarily in the lateral dimension in the majority of OSA patients (Schwab et al. 1997). Furthermore, treatment with CPAP, weight loss, or mandibular advancement all show increases in the lateral pharyngeal dimensions (Schwab et al. 1997). There are many potential causes of lateral wall thickening in OSA patients. First, as shown in both humans and rodent models, obesity is a major contributor to airway compression through increased area and volume of pharyngeal fat deposits (Brennick et al. 2009). This excess fat deposition has also been observed under the mandible and within the tongue, soft palate, or uvula. Obesity also gives rise to excess fat-free muscular tissue, thereby increasing the size of many upper airway structures and compressing the lateral airway walls. In children with OSA, tonsillar hypertrophy and adenoid hypertrophy form the major anatomical contributors to airway narrowing (Brennick et al. 2009).
24.3.1.4 Obesity and Lung Volume
Obesity also contributes indirectly to upper airway narrowing, especially in the hypotonic airway present during sleep, because lung volumes are markedly reduced by a combination of increased abdominal fat mass and the recumbent posture. In turn, the reduced lung volume reduces the “tug” on the trachea induced by the traction exerted via mediastinal structures by negative intrathoracic pressures and by the diaphragm descent, thereby further increasing the thickness of the lateral pharyngeal walls and narrowing the airway.
24.3.1.5 Airway Edema and Surface Tension
Surface tension of the liquid lining the mucosa affects collapsibility of the upper airway in the same way as it has been well documented in the lung’s airways. A higher surface tension in the upper airway wall of OSA patients has been reported using a method that quantifies surface tension as the force required to separate two surfaces bridged by a droplet of the liquid under study. Furthermore, in limited studies, surfactant therapy in OSA patients was shown to significantly reduce airway collapsibility and improve apnea-hypopnea index (AHI) by 20–30 % (Gleadhill et al. 1991).
24.3.1.6 Obesity, Leptin, and Inflammation
Central, or visceral, obesity is associated with the greatest risk for OSA (Shinohara et al. 1997). This suggests that factors other than pure mechanical load may contribute to the pathogenesis of respiratory disturbances during sleep. The concept is now emerging that visceral fat depots, which represent a rich source of humoral mediators and inflammatory cytokines, can impact on neural pathways associated with respiratory control (Schwartz et al. 2008). Perhaps the most well-studied adipocyte-derived factor affecting respiratory control is leptin, which was initially determined to have a primary role of binding to receptors in the hypothalamus to reduce satiety and increase metabolism. Leptin can also act as a respiratory stimulant, and impairment of the leptin signaling pathway, as occurs in leptin-resistant or leptin-deficient states of obesity, causes respiratory depression in mice and is associated with obesity hypoventilation syndrome in humans. Even though obesity and OSA are associated with elevated circulating levels of leptin, if centers in the brain impacting on respiratory control act in a similar leptin-resistant manner to hypothalamic regions controlling appetite and metabolism, then impaired leptin signaling in the CNS may contribute to respiratory depression as predicted in murine studies.
In addition to respiratory control, animal studies show that leptin is also critical in lung development and affects the distribution of muscle fiber types in the diaphragm. However, as yet there is no direct evidence that impaired leptin signaling can impact on the control of respiratory muscles of the upper airway, although it may play a role in nocturnal hypoventilation, particularly in REM sleep where respiration is markedly depressed in leptin-deficient mice (Tankersley et al. 1999).
24.3.2 Mechanical Determinants of Upper Airway Patency
Mechanical determinants of airway caliber of the human pharynx in sleep are similar to those regulating caliber of any collapsible tube (Schwab et al. 2005). Other well-known biological examples in respiratory physiology include intrathoracic airway collapse upon forced exhalation, collapse of pulmonary capillaries in the lung apex, and collapse of alae nase at high inspiratory flow rates. A Starling resistor model developed by Schwartz and colleagues consists of a collapsible tube with a sealed box interposed between two rigid segments (Gold and Schwartz 1996). The critical closing pressure (P crit) of the passive airway is defined as the pressure inside the airway (P in) at which the airway collapses. The pressure gradient during airflow through the system is defined by Pupstream and P crit and remains independent of Pdownstream. Therefore, with increasing P crit, as the differential between Pupstream and P crit decreases, inspiratory airflow limitation will eventually develop, and when the P us falls below P crit, complete airway occlusion occurs (Fig. 24.2). Effective therapy for sleep apnea requires that the P us to P crit pressure differential be widened, and this can be accomplished by either:
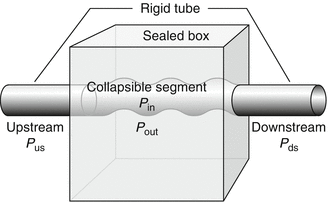
1.
An increase in P us with appropriate amounts of CPAP applied at the airway opening, or
2.
By decreasing P crit via either reducing the collapsing pressures on the airway (e.g., weight loss or alteration of cranial-facial anatomy or increasing lung volume) or by augmenting “active” neuromuscular control of airway tone (Gold and Schwartz 1996)
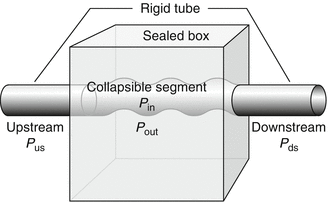
Fig. 24.2
Starling resistor model of obstructive sleep apnea. In the Starling resistor model, the collapsible segment of the tube is bound by an upstream and downstream segment with corresponding upstream pressure (P us ), downstream pressure (P ds ), and upstream resistance and downstream resistance. Airway occlusion occurs when the surrounding tissue pressure (P out ) (comprised of pharyngeal muscles and pharyngeal and submucosal fat, mucosal edema, etc.; see Sect. IIIC) becomes greater than the intraluminal pressure (P in ), resulting in a transmural pressure of zero. In this model of the upper airway, P us is atmospheric at the airway opening, and P ds is the tracheal pressure. The critical closing pressure of the collapsible airway (P crit) is represented by P in. When the P crit is significantly lower than P us and P ds, flow through the tube occurs. When P ds falls during inspiration below P crit, inspiratory airflow limitation occurs and is independent of further decreases in P ds. Under this condition, the pharynx is in a state of partial collapse, and maximal inspiratory airflow varies linearly as a function of the difference between P us and P crit. Finally, when P us falls below P crit, the upper airway is completely occluded
24.3.3 Neuromuscular Control of Upper Airway Dynamics in Sleep
Clearly the effects of airway anatomy on airway collapsing pressure in a hypotonic airway are a critical determinant of obstructive apnea. However, several lines of evidence also support neuromuscular factors as significant determinants of airway collapsibility in sleep. First, tonic and phasic EMG activities of pharyngeal airway dilator muscles (genioglossus and tensor palatine) are progressively reduced from wakefulness to NREM to REM sleep and further inhibited coincident with the “phasic” eye movement events in REM. This powerful effect of state has been adequately documented in tracheostomized animal models and recently has been demonstrated in OSA patients in whom the potentially confounding, compensatory responses to sleep-induced changes in upper airway resistance, negative pressure, PaCO2, and respiratory motor output were controlled through the use of either CPAP or positive pressure controlled mechanical ventilation (Lu et al. 2006). These state effects on the neuromuscular control of the upper airway likely explain, along with reductions in lung volume, why P crit is never positive in the waking state, even in OSA patients.
Second, neuromuscular factors also play a significant role in the dynamic breath-to-breath and intrabreath regulation of upper airway caliber, through changes in proprioceptive and chemoreceptor feedback. During inspiration, the passive pharynx narrows as intraluminal pressure is progressively reduced because of energy lost in overcoming frictional airway resistance and increases in flow velocity secondary to the Bernoulli effect operating in a reduced lumen size (Schwab et al. 2005). This collapsing effect of a reduced luminal pressure is opposed during inspiration by a reduction in dynamic compliance, i.e., collapsibility, of the airway achieved via reflex activation of pharyngeal dilator muscles. In turn, the reflex activation occurs in response to negative pressure airway mechanoreceptors located principally in the larynx and to a lesser extent in the superficial layers of the pharyngeal wall, with their afferent projections located in the superior laryngeal nerve, and also in glossopharyngeal and trigeminal nerves (Schneider et al. 2002). Large changes in negative pressure in the isolated upper airway trigger a dual protective reflex, which restores airway patency by both activating airway dilators (to reduce airway compliance) while inhibiting diaphragm EMG activity (which minimizes intraluminal negative pressure) (Fig. 24.3). Vagally mediated feedback influences on laryngeal, tongue, and hyoid muscle via pulmonary stretch receptors also protect against airway collapse as the rate of lung inflation is slowed in the face of increased airway resistance, thereby reflexly activating upper airway motor neurons.
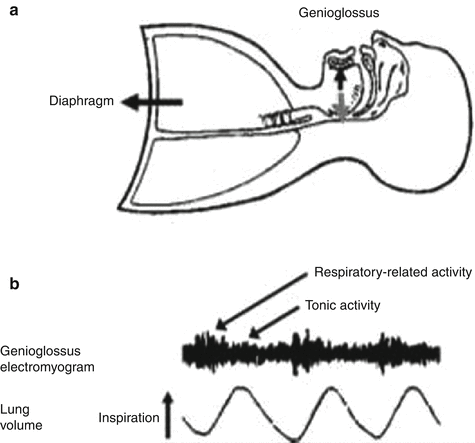
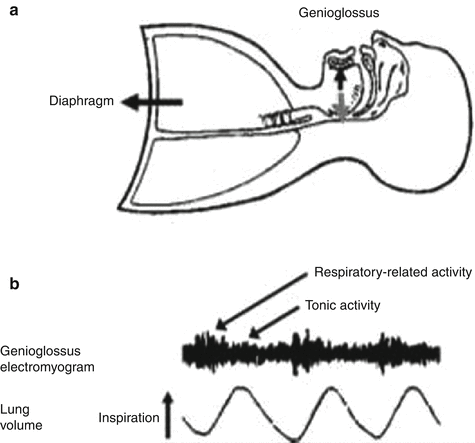
Fig. 24.3
(a) Contraction of the diaphragm and chest wall muscles leads to the generation of subatmospheric pressures in the thoracic cavity and airflow into the lungs. For effective lung ventilation, however, this airflow must pass through an open upper airspace. Activation of the pharyngeal muscles, such as the genioglossus muscle of the tongue, helps keep the upper airspace open for effective passage of air. Pharyngeal muscle activation acts to enlarge the airspace. Importantly, reduced pharyngeal muscle tone in sleep can reduce the size of the upper airspace and even promote complete airway obstruction. This tendency for airway collapse is exacerbated by increased weight of the tongue or neck (e.g., caused by obesity), an already anatomically narrow upper airway (e.g., caused by adenotonsillar hypertrophy), or the supine sleeping position. (b) The pharyngeal muscles exhibit respiratory-related activity superimposed upon a background of tonic activity. The background tonic muscle tone contributes to baseline airway size and stiffness. The increased pharyngeal muscle activity during inspiration enlarges and further stiffens the airspace to resist the subatmospheric collapsing pressures generated during inspiration
Finally, chemoreceptor influences also have substantial effects on upper airway muscle recruitment, and in the case of CO2, upper airway motor neurons relative to phrenic motor neurons have been shown to have a substantially higher threshold for inhibition (via hypocapnia) and activation (via hypercapnia) (Weiner et al. 2002).
In summary, the evidence to date supports important roles for both anatomical and neural control of dilator muscles to the regulation of upper airway caliber in the sleeping human. The relative contributions of these factors will vary widely among and within individuals with, for example, patterns of fat deposition on the one hand and neurochemical sensitivity for dilator muscle recruitment on the other.
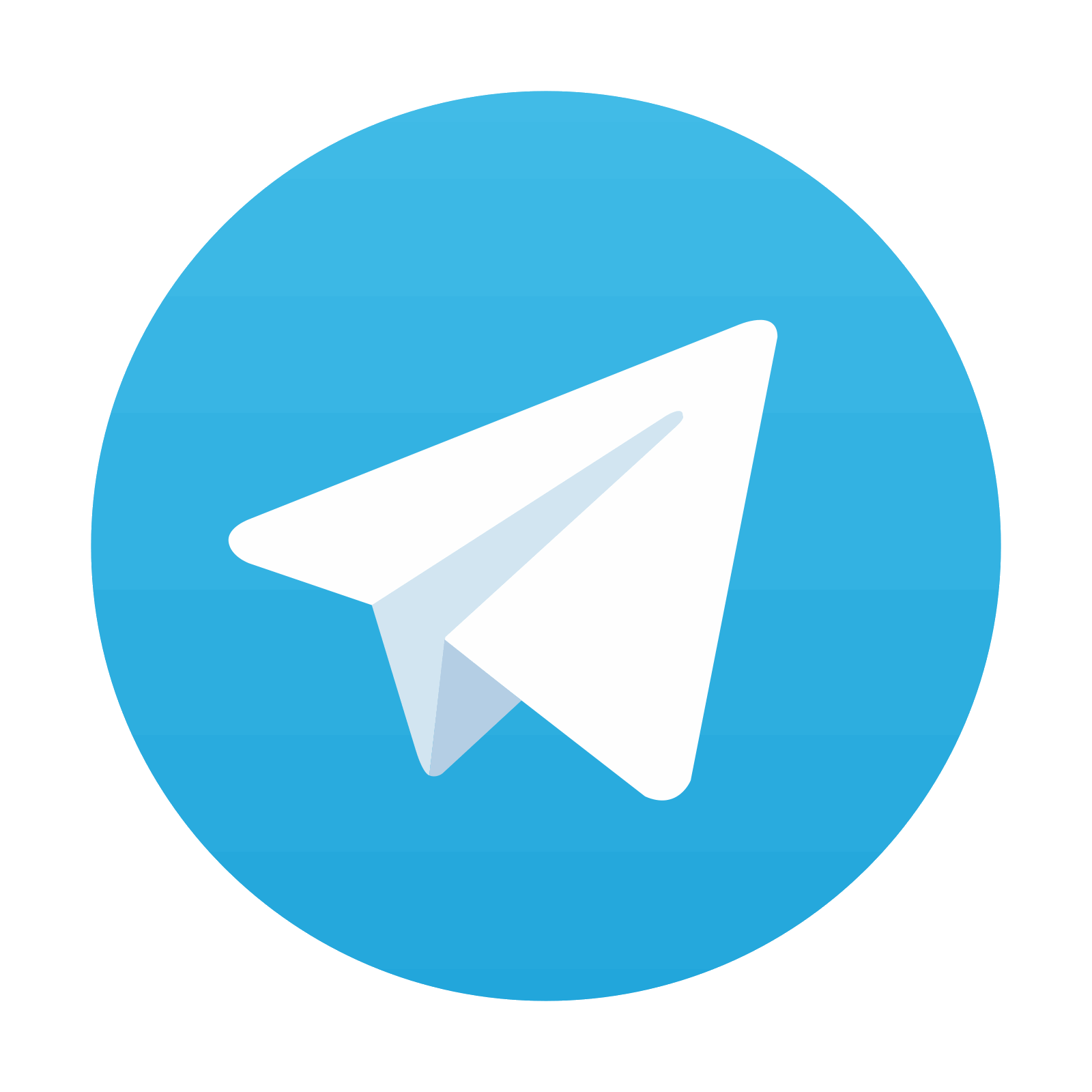
Stay updated, free articles. Join our Telegram channel
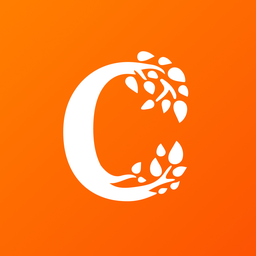
Full access? Get Clinical Tree
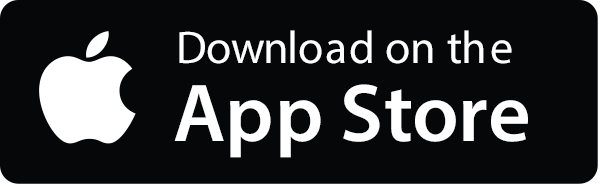
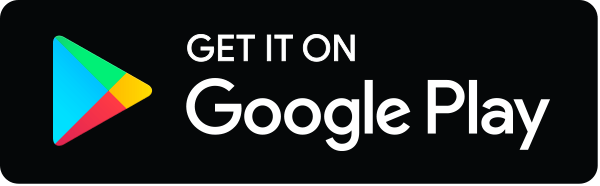