Fig. 3.1
The “hydraulic” theory of the orbital floor fracture (citation from Della Rocca [52]). See explanation in the text: It is an interesting fact that, contrary to the established opinion, a tennis ball striking the eye does not cause a fracture although it may damage the globe. The deformation of a hollow ball during the contact and the suction effect when the ball bounces off the periorbital area presumably dampen the increase in intraorbital pressure. A fracture can be caused by the impact of an injuring agent incapable of transitory deformation (e.g., a fist)
There are fewer supporters of the buckling theory formulated by R. Le Fort [53] who believe that wavelike deformations transmitted from the infraorbital rim is the main mechanism of blow-out fracturing of the orbital floor [54]. Depending on the direction of the force vector, the orbital floor (mostly its interior half) undergoes either a horizontal or rotational deformation [55]. The fracture area will be maximal when an injuring agent is moving upward at an angle of 30° to the infraorbital rim (Fig. 3.2) [56].


Fig. 3.2
The buckling theory of the formation of an orbital floor fracture: (a) The dorsal view of the inferior wall of the right orbit. The main mechanisms of blow-out fracturing of the orbital floor are wavelike deformations (shown with dashed lines) that are transmitted from the intraorbital rim to the orbital floor. (b, c) A horizontal impact (I1) causes less significant deformation (D1) compared to impact (I2) that is parallel to the orbital floor plane (D2)
The kinetic energies required to damage the orbital floor via wavelike deformation and hydraulic shock are almost identical [57–60], but localization and extension of the “hydraulic” and “buckling” fractures differ considerably [61]. Cadaveric studies of the orbits demonstrated that fractures caused by wavelike deformation mostly localize in the anterior half of the orbital floor, do not affect the medial wall, and are not accompanied by soft tissue entrapment in the bone defect area. The hydraulic mechanism causes significantly more extensive fractures affecting both the entire orbital floor and the medial orbital wall and results in soft tissue prolapse and enophthalmos [60, 62].
Some authors [17, 52, 60] believe that both mechanisms contribute to fracture formation and it is fundamentally wrong to contrapose them. The variety and complexity of orbital fractures sometimes can be explained only by the simultaneous presence of both trauma mechanisms and with predominance of one over the other giving the individual pathological appearance in each specific case [62].
Another mechanism of fracture formation has been propose before: “pressing against” the orbital floor by the equator of the eyeball abruptly deformed at the moment of globe trauma. Some authors support this hypothesis of Pfeiffer [63] even today [62, 64]. However, cadaver studies of the orbits relying on advanced mathematical models have demonstrated that it is soft tissues adjacent to the orbital floor that act as a direct injuring agent rather than the globe itself [65].
An isolated blow-out fracture of the orbital floor requires less energy compared to the damage of the medial wall or formation of an inferomedial fracture [65].
This fact, which may seem to be unreasonable at first glance, is well known among clinicians and was explained by Takizawa et al. [66]. The authors used experiments and subsequent computer simulation to demonstrate that both the thickness and the contour (profile) of orbital walls play a crucial role. In particular, the arch-shaped orbital roof is much more resistant to deformation as compared to the virtually planar floor, which can be deformed and fractured more easily. The medial wall is even thinner but it is reinforced by ethmoidal air cells. Hence, the fracture of the medial wall requires greater mechanical energy compared to that of the orbital floor [67, 68].
Such anatomical structures as the inferior orbital fissure, the infraorbital groove, and infraorbital canal [66], as well as reflexive contraction of the m. orbicularis oculi and presence of a large air-bearing cavity [17, 27] under the orbit, also facilitate the damage secondary to trauma to the orbital floor. The rarity of orbital floor fractures in children younger than 7–8 years is caused by underdevelopment of the maxillary sinus and continuing growth of the orbit [1, 69–72]. Even if a fracture is formed, it requires surgical management less often compared to adults [71].
3.3.2 Classification of Blow-Out Fractures of the Orbital Floor
According to radiologic classification proposed by Fueger et al. [73], blow-out fractures are subdivided into six main types, with some of them containing subtypes:
1.
Classical blow-out fracture: a low-energy fracture of the medial half of the orbital floor medial to the infraorbital canal (Fig. 3.3). This variety is the most common because this is the weakest area of the orbital floor [74] and is observed in 50 % of cases [75].


Fig. 3.3
Typical forms of orbital floor fractures according to Fueger et al. [73]: (a) Classical fracture, confined to the orbital lamina of the ethmoidal bone and infraorbital canal. The inferior orbital fissure often acts as the posterior border. (b) In most cases, a coronal CT scan shows the bony “cusp” with its base oriented toward the nose (an arrow), which illustrates the origin of the term “open-door fracture.” (c, d) Less frequently, the fracture is formed by two bony “cusps” (an arrows)
2.
Fracture involving the infraorbital canal (Fig. 3.4a, b).


Fig. 3.4
Typical forms of orbital floor fractures: (a) Schematic representation of a fracture involving the infraorbital canal. Anterolateral view. (b) Coronal CT scan. Thick and thin arrows show the bony “cusp” and the infraorbital canal, respectively. The canal is adjacent to the belly of the inferior rectus muscle (shown with an asterisk). (c) Fracture of the inferior and medial walls (inferomedial fracture). Anterolateral view. (d) Coronal CT scan. Prolapse of orbital tissues into the maxillary sinus is indicative of orbital floor fracture; discontinued contour of the medial wall and opacity of ethmoidal air cells are indicative of medial wall fracture
3.
Inferomedial fracture, i.e., a fracture of the inferior and medial walls. A number of authors [75, 76] reported that this middle-energy variant of trauma accounts for 20–40 % of all orbital floor fractures (Fig. 3.4c, d). Thus, the two most frequent orbital floor fractures are the classical and inferomedial ones. The share of all other variants is less than 10 % of cases.
4.
Total fracture of the orbital floor (Fig. 3.5). Propagation of the fracture lateral to the infraorbital canal is typically caused by the effect of an injuring agent having an appreciably high kinetic energy, which results in fracturing of the entire orbital floor.


Fig. 3.5
Total fracture (i.e., the fracture spreading outward from the infraorbital canal): (a, b) Schematic representation of the fracture in the anterolateral (a) and coronal (b) views. (c) Total saucer-like fracture (arrow); (d) comminuted total fracture (arrow); (e, f) total open-door fracture (arrow)
5.
Atypical forms of blow-out fractures:
(a)
Rectangular fracture
(b)
Triangular fracture
(c)
Stellate fracture
6.
Linear fractures of the orbital floor without fragment displacement:
(a)
Y-shaped fracture
(b)
Lateral linear fracture (Fig. 3.6)


Fig. 3.6
Atypical forms of orbital floor fractures (on the example of left orbit, anterolateral view) (citation from Fueger et al. [73]): (a) Rectangular fracture running parallel to the infraorbital canal. (b) Triangular fracture. (c) Stellate fracture. (d) Y shaped. (e) Lateral linear fracture connecting the inferior orbital fissure with the infraorbital rim (Cited from Greenwald et al. [77]). (f) Coronal CT of the fracture of the lateral half of the orbital floor (shown with arrows)
3.3.3 Diagnosis of Blow-Out Orbital Floor Fractures
Diagnosis of the blow-out orbital floor fracture is often facilitated by the presence of typical complaints. Diplopia during vertical eye movement is the key finding and is observed in 58 % of patients [78].
The primary position of gaze and changes in diplopia intensity during eye movement help to localize the inferior rectus entrapment site.
Lerman [79] formulated rather interesting rules related to diplopia in patients with blow-out fractures:
1.
Thus, if diplopia is aggravated with upward gaze and improved with downward gaze, while the eyeball in the injured orbit is slightly deviated downward in the primary position of gaze, the inferior rectus muscle is entrapped in the pre-equatorial zone (Fig. 3.7).


Fig. 3.7
Vertical eye movement problems (for the left eye) when a fracture localizes in the pre-equatorial zone (the equator is shown in (d) with a black line). The left eyeball is slightly deviated downward in primary position of gaze (b); diplopia is aggravated with upward gaze (a) and improved with downward gaze (c). Black arrows – direction of gaze (citation from Lerman [79])
2.
If limited mobility and diplopia have the same intensity both for the upward and downward gaze, while the eye is oriented centrally in the primary position of gaze, the muscle is entrapped in the equator of the eyeball (Fig. 3.8).


Fig. 3.8
Vertical eye movement problems (for the left eye) when a fracture localizes in the equatorial zone of the left eyeball (the equator is shown in (d) with a black line). The left eyeball is oriented centrally in primary position of gaze (b); limited mobility and diplopia have the same intensity both for upward (a) and downward (d) gaze (citation from Lerman [79])
3.
Finally, if diplopia is aggravated with downward gaze and improved with upward gaze, while the eyeball is slightly deviated upward in the primary position of gaze, the inferior rectus muscle is entrapped postequatorially4 (Fig. 3.9).


Fig. 3.9
Eye movement problems when the fracture (shown with arrows on the CT scan) localizes behind the equator of the eyeball (the equator is shown in (d) with a black line). The eyeball is slightly deviated upward in primary position of gaze (b); diplopia is aggravated with downward gaze (a) and improved with upward gaze (c) (citation from Lerman [79]). Posterior fractures of the orbital floor are accompanied by the most severe and persistent diplopia caused by the entrapment of posteroinferior portions of the adipose body of the orbit, which are permeated by numerous connective tissue intersections that are interwoven into the sheaths of extraocular muscles, in the bone defect
Paralysis of the inferior rectus complex [80], displacement of the inferior muscular complex into the fracture zone [81], and the abnormal angle of attachment of the dislocated inferior rectus muscle to sclera [82] are considered to be possible reasons for upward deviation of the eyeball (Fig. 3.10).


Fig. 3.10
A tentative mechanism of eyeball tropia in patients with posterior fractures of the orbital floor: (a) Schematic representation. (b–d) A CT scan contains an extensive (stretching up to the posterior wall of the maxillary sinus) defect (shown with an asterisk) or depression (shown with an arrow) of the orbital floor. The inferior rectus muscle is displaced downward. It should be mentioned that the muscle is not entrapped in the fracture zone and the traction test will be negative prior to surgery (Fig. 3.14). Hypofunction of the inferior rectus muscle is caused by other reasons: attachment to the sclera at an angle more obtuse than the physiological one (up to the right angle); displacement of the muscle origin from the orbital apex to the point where the muscle is “detached” from the orbital floor displaced downward; impaired motility of the muscle as it passes over a sharp edge of the defect, thus the effect of faden operation (muscle relaxation by suturing its belly to the sclera 13 mm away from its attachment site) is imitated
Complaints of hypoesthesia in the distribution of the infraorbital nerve occur in 70 % of patients [84] (Fig. 3.11). The combination of neurological impairments with vertical diplopia and enophthalmos allows one to almost for certain make a clinical diagnosis of orbital floor fracture [85].


Fig. 3.11
The region innervated by the infraorbital nerve: 1 nasal branch (nasal skin and septum), 2 labial branch (upper lip skin and the oral mucosa), 3 palpebral branch (skin and conjunctiva of the lower eyelid)
A meticulous history to discover the mechanism of trauma is helpful for making the proper diagnosis. One should pay attention to two major factors that determine the clinical presentation of a fracture: the size of an injuring agent and the energy component of the trauma. If the surface area of a blunt object is smaller than the size of the orbital opening, the patient may have rupture of the sclera. If the size of the injuring body is larger than that of the orbital opening, two alternative outcomes are possible. If the injuring object has a relative low speed and, therefore, low kinetic energy, the low-energy blow-out orbital floor fracture occurs. A rather strong impact results in a middle-energy trauma that may combine a fracture of the infraorbital rim and the orbital floor (Fig. 3.12a–d) [49]. Finally, a large injuring object with high kinetic energy may cause a fracture of the orbital rim, orbital floor, and other facial bones. And if enough energy is present, panfacial fractures may occur (Fig. 3.12e, f) [44]. These situations are usually caused by car accidents. Thus, the analysis of the traumatic circumstances is of practical importance as it allows one to predict the type of injury and question the signs of periocular edema and hematoma found during the primary examination that may hide a more serious underling injury.


Fig. 3.12
Middle- and high-energy orbital fractures: (a–d) Middle-energy fracture of the infraorbital rim and inferior wall of the orbit (bone fragments are shown with arrows)
An objective examination starts with external inspection. Possible orbital floor fracture is indicated by such findings as pronounced palpebral edema and hematoma, subconjunctival hemorrhage, and chemosis of the bulbar conjunctiva (Fig. 3.13a, b) [2, 86].


Fig. 3.13
Clinical presentation of orbital floor fracture (white line demonstrates movement restriction): (a, b) Periorbital ecchymosis, subconjunctival hemorrhage, palpebral edema, and hematoma. (c) Narrowing of the left orbital fissure that is clearly seen by comparing the positions of the lower eyelid on both sides. (d) Left-sided enophthalmos manifesting as deepening of the upper eyelid crease. (e, f) Ipsilateral restriction of supraduction (upward movements of the eyeball)
The concomitant findings and symptoms of orbital floor fractures are listed in the order of decreasing incidence: periorbital ecchymosis (75 %), diplopia (50–60 %), subconjunctival hemorrhage (40 %), and enophthalmos (33 %) [2, 9].
It is commonly believed that if a patient has these findings, it is possible to reliably assess the eyeball position in the orbit (in three views) and scope of its movements only 2–3 weeks after the injury [87], since reactive edema and hematoma of orbital soft tissues may disguise enophthalmos up to 3 mm in size5.
Axial dystopia (eno- or exophthalmos) is determined with respect to the relatively healthy eye using a Hertel/Krahn exophthalmometer. A basis is selected so that the supports are tightly pressed against the anterior surface of the lateral orbital rims. An ophthalmologist uses his/her left eye to assess the protrusion of the patient’s right eye and vice versa. When assessing the patient’s right eye, the ophthalmologist’s left eye is supposed to be open, while the patient looks at the ophthalmologist’s closed right eye. The ophthalmologist uses his/her left eye to converge parallel lines into one line in a mirror and evaluates corneal protrusion using the scale drawn on the mirror [89].
Exophthalmos in the presence of injury to the orbital floor is possible only during the acute phase of trauma and is caused by edema and/or hematoma of orbital tissues. Globe retraction (sunken eye) is a typical symptom of blow-out fracture (Fig. 3.13c, d). Enophthalmos is indicative of increased orbital volume, which is typical of blow-out fractures with significant displacement of bone fragments (the classical, inferomedial, and total ones).
One to two millimeter dystopia is considered to be mild; 3–4 mm dystopia is regarded as moderate; enophthalmos greater than 5 mm is considered to be pronounced [90].
Yab et al. [88] analyzed CT scans of patients with orbital fractures and found that enophthalmos remains ~1 mm if the orbital volume increase is less than 2 mL.
The degree of enophthalmos subsequently increases in proportion to increasing orbital volume; however, it is never greater than 4 mm for an isolated orbital floor fracture.
Vertical dystopia (hypoglobus) is assessed with respect to a horizontal line running through the center of the pupil of the healthy eye [91, 92]. Eyeball prolapse is usually indicative of the extensive orbital floor fracture. Sporadic cases of dislocation of the eyeball into the maxillary sinus are the most marked variants of hypoglobus.
Lateral dystopia (in the frontal view) is measured by comparing the distance between the midpoint of the nasal bridge and the nasal portion of the limbus. The difference between the healthy and the injured sides is indicative of concomitant fracture of the medial orbital wall [89].
An analysis of eye movements is the next step of examination. The position of the eyeballs in primary gaze is first evaluated followed by assessment of ocular excursions across the horizontal and vertical meridians. The ocular motility in the six main directions of gaze is then inspected: rightward, leftward, upward and outward, upward and inward, downward and outward, and downward and inward. Total restriction of ocular motility is usually indicative of orbital edema or hematoma. Ipsilateral reduction of supraduction or infraduction is a diagnostically valuable finding (Fig. 3.13e, f). One should bear in mind that obvious restrictions of ocular motility are rather rare [93]. An induced diplopia test based on evaluation of the relative spatial position of the images produced by the normal and deviated (injured) eye is conducted to detect less evident ocular motility disorders.
Diplopia is induced by placing a red lens in front of an eye. This allows one to simultaneously find out which of the double images belongs to the right and left eye, respectively. The distance between an ophthalmologist and a patient facing one another should be 1.5–2 m. The ophthalmologist holds a flashlight and moves it rightward, leftward, upward, and downward, as well as in intermediate directions. The patient is asked whether he/she sees one or two lights. If the patient sees two lights, he/she is asked to tell how they are oriented with respect to each other, what the distance between them is, and when the distance increases or decreases.
The results are assessed using the following rules: (1) the injured muscle corresponds to the eye producing an image located further from the median horizontal or vertical line; this image is referred to as the ghost image; (2) the ghost image is always projected toward the paralyzed muscle; hence, homonymous diplopia emerges when abductor muscles are affected, while heteronymous diplopia is caused by damage to the adductor muscles; and (3) the distance between double images increases as gaze moves toward the injured muscle.
Differential diagnosis between the main reasons for ocular motility disorders (paresis of the oculomotor nerve branchlet or muscle entrapment in the fracture area) is performed using the traction test (forced duction) [94, 95].
The patient is asked to look at his/her left arm outstretched in the direction of suspected displacement of the eyeball (i.e., upward)6. After epibulbar anesthesia, the limbal conjunctiva at 6 o’clock meridian is fixed with corneal (colibri) forceps, and the eyeball is displaced in a direction opposite to the vector of traction of the inferior rectus that is suspected to be entrapped (i.e., upward). It is important that the eyeball is not pressed against the orbit, which may create an illusion that there is normal ocular motility with muscle entrapment [83].
A positive traction test is the impeded passive upward displacement of the eyeball, while its downward motility remains normal. According to clinical observation, ocular motility is limited both in up- and downward directions. The positive traction test indicates that either the inferior rectus or the inferior rectus and inferior oblique muscles are entrapped in the fracture area. In a number of cases, only the inferior portion of the anterior suspensory system is entrapped (Fig. 1.35); however, its close connection with the muscles also causes diplopia [94, 96] and requires surgical intervention.
A negative traction test (unimpeded passive upward displacement of the eyeball) is observed in 18 % of cases [17, 97, 98] and is indicative of rectus superior paralysis (Fig. 3.14) [83] or injured muscle (contusive edema, hematoma, or muscle detachment from the sclera) [99].


Fig. 3.14
The traction test procedure (citation from von Noorden [83]; Della Rocca [52]): (a) After epibulbar anesthesia, the limbal conjunctiva at 6 o’clock position is immobilized with corneal forceps. (b) The eyeball is displaced upward. (c) Positive traction test corresponds to impeded passive upward displacement of the eyeball (shown with a curly arrow), which is indicative of entrapment of the inferior rectus muscle (shown with dark) in the fracture area. (d) Negative traction test corresponds to unimpeded passive upward displacement of the eyeball (shown with a curved arrow), which is indicative of paralysis of the levator (the superior rectus muscle shown with dark)
The so-called generation test (muscular effort generation test) is used in this instance [100]. After epibulbar anesthesia, the limbal conjunctiva at 6 o’clock meridian is fixed with corneal forceps, and the eyeball is held in the primary gaze direction. The patient is asked to look in the direction of action of the examined inferior rectus (i.e., downward). If innervation is retained, the ophthalmologist will feel the effect of the muscle trying to move the eyeball down (the positive test). The negative result of the test cannot be easily and unambiguously interpreted as it can be caused both by paralysis of the oculomotor nerve and by injury to the muscle. In most cases, the reason for the negative test is secondary to muscular dysfunction that typically disappears within 1–2 weeks. Chronic oculomotor disorders show that they are of neurogenic origin.
The contraindications to performing the traction test include acute pain, blepharospasm, orbital hematoma, or tissue edema because the risk of obtaining false-positive results is high [52].
Despite the fact that a blow-out fracture partially absorbs increased intraorbital pressure, these traumas are accompanied by various eye injuries in 30–40 % of cases [21, 28]. Severe intraocular injury occurs rather frequently (20–30 %) [12, 33, 101]. Tong et al. [9] reported that 40 % of these injuries are scleral ruptures. In 38 % of cases, orbital floor fractures are accompanied by injuries affecting structures other than facial bones (first of all, cranial and cerebral traumas). The risk of suffering an eyeball trauma for blow-out fracture is 2.5 times as high as that for zygomatic orbital fracture [33]. It is always mandatory that the integrity of the eyeball is established prior to any orbital floor reconstruction [102]. Unfortunately, traumas of the eyeball and periorbital area often go unnoticed at the primary inspection of patients with midfacial fractures [29].
The first parameters to be assessed in a newly admitted injured patient are visual acuity and pupillary responses [103].
Complete evaluation of the visual system in the emergency department is often unfeasible. Accurate evaluation of visual acuity when the patient is examined at the hospital may be caused by a variety of reasons: an altered mental state either from trauma or intoxication, the absence of corrective lenses including the loss of contact lenses, tears admixed with blood, pain, poisoning, fear, or anxiety, which may prevent the patient from focusing. While the absolute accurate assessment of visual acuity may not contribute to the treatment decision making at that time, it is very important to document the reason why the complete evaluation was not possible. However, the finding of profound loss of vision clearly indicates that the visual pathway has been seriously damaged.
In the absence of central vision, either real or apparent, an ophthalmologist should perform a light sensitivity test comparing the light sensitivity of the traumatized eye to the normal eye. The test for distinguishing the red color is performed to assess the status of the optic nerve. An unfavorable prognostic factor is if the patient perceives the tested object (either a distal phalanx of the index finger or a red cap from the vial of a mydriatic agent) as having an orange or brownish tint.
Regardless of the patient’s consciousness, the pupillary status, and evaluation for a relative afferent pupillary defect (RAPD) must be done. If the patient is unconscious or uncooperative, this may be the only test of the visual system that can be done. Particular attention should also be given to the shape and size of pupils [12].
Corectopia or a peaked pupil is a sign of penetrating eye injury or scleral rupture.
When performing evaluation of the pupils, one should bear in mind that pupil diameter does not closely correlate with visual acuity, because the pupil size depends on interaction between sympathetic fibers of the ophthalmic division of the trigeminal nerve (n. V1) and parasympathetic fibers located in the inferior branch of the oculomotor nerve (n. III).
As a result, a blind eye may have a normal pupil diameter, and vice versa, a patient with the maximum degree of mydriasis may have high visual acuity.
Pupillary responses to the bright light are then analyzed. The afferent pupillary defect, or the Marcus Gunn pupil (impaired direct pupillary response to the light with the retained consensual response), confirms the presence of optic nerve trauma. The pupil in this case exhibits a paradoxical response to the light beam being rapidly moved from a healthy eye to the injured one. The pupil of the injured eye does not constrict under direct exposure to light; instead, it widens as it loses the direct response due to the impairment of the afferent portion of the pupillary reflex arc.
The next stage involves examination of the central visual field using the Amsler grid (Fig. 3.15) and estimation of visual field borders. After that, the anterior segment is examined using a flashlight. The final stage of examination is ophthalmoscopy of the fundus under drug-induced mydriasis. The pharmacological effect on the pupil and meticulous description of the initial state of the pupil must be reported in patient’s medical history prior to dilation. Unless necessary, the pupil of the contralateral healthy eye should not be dilated.


Fig. 3.15
Amsler grid for examining the central (20°) visual field: The 200 × 200 grid consists of squares with a side length of 5 mm formed by intercepting vertical and horizontal lines. The fixation point is placed in the center of the grid. A patient looks at the fixation point and sees the lines either as smooth and uniformly colored or distorted and partially obscure depending on his/her retinal status. The patient draws the pattern he/she sees on the Amsler grid
The only symptom specific of orbital floor fracture that can be detected by visual inspection of the eyeball is pupil dilation to 5–8 mm persisting from several weeks to several months after the trauma. The pupil does not respond to light but is narrowed after pilocarpine instillation, which allows one to differentiate between this pathology and contusion-induced mydriasis7 [105].
The obvious CT signs of the orbital floor fracture include muscle entrapment in the fracture area and extensive prolapse of the orbital adipose tissue into the maxillary sinus (Fig. 3.16a, b).


Fig. 3.16
CT signs of the orbital floor fracture: (a) An extensive bone defect with displacement of a bone fragment (shown with an arrow) into the sinus. (b) Prolapse of the orbital fat entrapped in the trapdoor fracture into the maxillary sinus (arrow). (c–e) Rounding of the normally flat belly of the inferior rectus muscle (shown with an arrow). The sign does not have a significant prognostic value in evident fractures (c) but is rather informative for small defects of the orbital floor (d, e). (f) Massive hemorrhage into the maxillary sinus, which facilitates diagnosis of a fracture with minimal displacement of bone fragments
The indirect signs of the fracture are as follows (Fig. 3.16c, d):
The muscle is adjacent to the seemingly uninjured orbital floor. The combination of this sign with the typical clinical presentation indicates that the sheath of the extraocular muscle and the surrounding connective tissue intersections in the area of linear fracture are subjected to trapdoor entrapment [95, 106, 107].
The missing inferior rectus muscle syndrome, when the muscle is entrapped in the bone defect area in patients with trapdoor fractures in such a way that it is imaged neither in the orbit nor in the maxillary sinus in coronal CT scans [108–110].
Rounding of the normally flattened belly of the rectus muscle that is clearly discernible on a coronal CT scan (Fig. 3.16c–e) [12, 111]. This indicates that the muscle is no longer supported by the bones and connective tissue [112, 113]. It was found in cadaver studies using orbits that when the fracture area is less than 1 cm2, rounding of the belly of the inferior rectus is caused only by periosteal rupture. This leads to a high risk of late enophthalmos and requires early surgical management. When the fracture area is 4 cm2, the belly becomes round even if the periosteum is not ruptured; however, the symptom is more marked in case of periosteal rupture [112].
If patient’s general status is serious and coronal CT scanning is unfeasible, one can use transantral endoscopy via the approach to the maxillary sinus using the Caldwell–Luc procedure. This can be performed under local anesthesia in hospital and is a very helpful method to evaluate and treat the patient [115].
3.3.4 Management of Blow-Out Fractures of the Orbital Floor
3.3.4.1 Indications for Surgery
Blow-out fractures that make a patient suffer neither functionally nor aesthetically do not require surgical management [116]. All other cases are managed surgically. Сonservative management or delayed surgery are not used any longer [117].
Management of the orbital floor fracture is aimed at restoring the original shape and volume of the orbit, repositioning its contents, and recovering ocular motility [117–119]. The formula for success consists in adequate exposure of the fracture area, clear visualization of its posterior edge, and compensation for the defect within its entire area [74].
Regardless of the fact that no prospective randomized studies focused on management of the orbital floor fractures have yet been conducted, much clinical experience has led to clear indications for surgery [120, 121].
The intervention needs to be early, single stage, and definitive. Indications for reconstruction of the orbital floor during the first 3 days after trauma include:
Early hypo- and enophthalmos indicating the total fracture of the orbital floor (Figs. 3.5 and 3.17a)
Fig. 3.17
Indications for reconstruction of the orbital floor: (a) Total fracture. (b) The volume of the injured orbit is significantly increased. (c–e) Orbital floor defect occupying a half of the orbital area in the coronal (c) and sagittal (d) views and on a 3D reconstruction (e); (f) entrapment of the inferior rectus muscle in the zone of the linear trapdoor fracture has a high rate of development of strangulation necrosis
In other cases of acute concomitant injuries of the orbit and midfacial area, the integrity of the orbit should be restored on day 3–9, when there is neither life hazard nor risk of vision loss or serious vision impairment [124]. More than two-thirds of American plastic surgeons perform this intervention within the first 14 days [84], while half of British surgeons operate on the orbital floor fracture 6–10 days after trauma [127].
Each of the criteria listed below or their combination is an indication for surgery:
Diplopia in the functionally crucial gaze directions (e.g., for downward gaze 10 (within 30° of the primary gaze direction) [12, 123] or for direct gaze outward) persisting for 2 weeks after trauma in patients with radiologically verified fracture and positive traction test [120].
An orbital floor defect larger than half of the area of the orbital floor (Fig. 3.17c) [87, 130–132]. This is associated with an increased risk of developing late hypo- and enophthalmos [120].
Enucleation with insertion of an orbital implant in patients with a concomitant extensive orbital floor defect needs to include osteoplasty as the final stage. Otherwise, the patient will develop an anophthalmic enophthalmos and hypoglobus (Fig. 3.18) [136, 137].


Fig. 3.18
MR image of an anophthalmic “enophthalmos” in a patient with a total orbital floor fracture: (a) Pronounced retraction of a cosmetic prosthesis on an axial MR image. (b) The coronal view shows the downward displacement of the orbital contents into the maxillary sinus. (c) Prolapse of orbital fat and orbital implant malposition that are clearly seen in the sagittal view
The intervention is regarded as early if it was performed in the acute phase of trauma, i.e., within the first 14 days [87, 138]. This term is considered to be optimal for reconstructing the damaged orbit and recovering ocular motility [16, 118, 130, 139, 140], although chances of success do not decrease if the repair is done later within a month after an injury [141].
A surgery performed between 3 weeks to 4 months after the trauma, during the so-called gray period, is regarded as delayed surgery [142]. In this case, the fused bone fragments still can be mobilized without performing osteotomy [17], and the prolapsed soft tissues can be detached from the fracture margin [143]. Finally, an intervention performed 4 and more months after the trauma and requiring osteotomy is considered to be late intervention [134, 144]. Neither good aesthetic nor functional results can be achieved in this period [140] as the soft tissues covering the fracture area are inevitably cicatrized after the trauma [145, 146].
3.3.4.2 Approaches to the Orbital Floor
An intervention should be performed under intravenous or endotracheal anesthesia avoiding pronounced arterial hypotension.
It is reasonable to start the surgery with a peritomy and placing an inferior rectus bridle suture (Fig. 3.19).


Fig. 3.19
Bridle suture placed on the inferior rectus
An approach to the orbital floor can be performed through a transcutaneous (infraorbital or subtarsal) or subciliary incision with various modifications, as well as through a transconjunctival incision (either with or without cutting the lateral palpebral ligament). Each of these methods has its own advantages and drawbacks [147–152].
The transcutaneous approach along the infraorbital rim (the infraorbital approach) (Fig. 3.20) is the technically simplest one; however, there is a high risk of complications from cicatrix formation. If the incision is displaced toward the temple, persistent lymphostasis may occur the large lymph node basins are transected. If the incision is displaced toward the nose, persistent lacrimation may result because of disruption of the lacrimal pump function [94].


Fig. 3.20
Infraorbital approach to the orbital floor: (a) Front view. (b) The incision profile (see explanations in text)
The subtarsal approach is recommended for elderly patients having folded skin of the lower eyelid [153]. This approach is a variant of the subciliary approach described below with formation of a skin–muscle flap.
After local subcutaneous anesthesia, an incision is made along the inferior edge of the tarsal plate on the subtarsal skinfold (Fig. 3.21). If the edema impedes its visualization, an incision is made 5–7 mm below the palpebral edge. The incision is started at a level of the inferior lacrimal punctum and ends 5–7 mm outward from the lateral margin of the orbital fissure. Skin is separated from the m. orbicularis oculi (2–3 mm in the downward direction) followed by its incision and exposure of the anterior surface of tarso-orbital fascia.


Fig. 3.21
Subtarsal approach to the orbital floor: (a) Front view. (b) The incision profile (see explanations in text)
The stepwise profile of the approach prevents coarse cicatrix formation; furthermore, innervation of the pretarsal and preseptal portions of the m. orbicularis oculi is not affected. The dissection is continued in the preseptal plane, i.e., along the orbital septum up to the infraorbital rim.
The subtarsal incision is associated with a lower risk of vertical shortening and eversion of the eyelid; however, it still leaves a visible cicatrix and the risk of lymphostasis is higher than that of the subciliary approach [12]. This approach cannot be used in young patients. The subtarsal approach is recommended for inexperienced oculoplastic surgeons.
The subciliary approach was proposed by J. Converse in 1944 (citation from [148]). After subcutaneous infiltration with lidocaine supplemented with adrenaline, an incision is made along the skinfold 1.5–2 mm below the ciliary edge and parallel to it11 starting from the medial corner of the orbital fissure (Fig. 3.22a, b). The skin is separated from the m. orbicularis oculi down to the inferior edge of the tarsal plate. At this level, the fibers of the m. orbicularis oculi are bluntly separated with exposure of the tarso-orbital fascia, which is subsequently transected near the infraorbital rim (Fig. 3.22c). An obvious advantage of the subciliary approach is that it provides sufficient visualization of the inferior and medial orbital walls and an almost indiscernible cicatrix is formed [154].


Fig. 3.22
Subciliary approaches to the orbital floor: (a, b) Front view. (c) The classical approach proposed by J. Converse (1944). (d) The “skin-only” procedure including formation of an isolated skin flap. (e) The “non-stepped skin–muscle flap” procedure. (f) Dissection of a “stepped skin–muscle flap”
The “skin-only” modification was proposed by aesthetic plastic surgeons in the late 1960s. A typical incision is made, and a skin flap is separated from the m. orbicularis oculi in the downward direction, down to the level of the infraorbital rim where the fibers of the m. orbicularis oculi, the tarso-orbital fascia, and the periosteum are subsequently separated (Fig. 3.22d). The drawbacks of this approach include possible skin flap necrosis and development of transient ectropion in up to 40 % of cases.
The Non-stepped Skin–Muscle Flap Procedure. Incision of the skin and m. orbicularis oculi 2 mm below the eyelash line is followed by a separation of the eyelid along the surface of the tarsal plate and tarso-orbital fascia up to the infraorbital rim where it is dissected along with periosteum (Fig. 3.22e). In order to prevent shortening of the lower eyelid, it is important that the periosteum is cut on the anterior surface of the infraorbital rim, i.e., several millimeters below the site where the tarso-orbital fascia is attached to the bone [155].
A “stepped skin–muscle flap” procedure has been proposed because a subciliary incision is sometimes complicated by denervation of the pretarsal portion of the m. orbicularis oculi. This may lead to atonic eversion of an eyelid with sclera exposure near the inferior limbus.
An incision is made 2 mm below the eyelash line and is followed by dissection of the skin from the m. orbicularis oculi for 2–3 mm downward and then dissection of the m. orbicularis oculi and exposure of the anterior surface of the tarso-orbital fascia below the tarsal plate. Separation is then performed along the tarso-orbital fascia up to the infraorbital rim. The fascia and periosteum of the orbital floor are incised within the same plane (Fig. 3.22f). As a result, the strip of pretarsal orbicularis muscle continues to maintain the proper position of the lower eyelid.
More recently, others [52, 156, 157] have refined the procedure. Local injection of anesthesia in the subconjunctival and subcutaneous layers of the lower eyelid will result in hydrodissection of the subconjunctival and the precapsulopalpebral space. A 5-mm-long horizontal incision of the lateral canthus and transection of the inferior crus of the lateral ligament are then performed (Fig. 3.23a–d). This procedure mobilizes the lower eyelid and facilitates making an incision along the inferior conjunctival fornix. The lower eyelid is pulled away with a Desmarres lid retractor; a Jaeger lid plate is used to push the eyeball deeper inside the orbit. The palpebral conjunctiva and the lower lid retractor are dissected 3 mm above the conjunctival fold along the entire eyelid to end slightly medially from the projection of the lacrimal point (Fig. 3.23e–g). Thorough hemostasis is performed using diathermy. The periosteum is dissected along the infraorbital rim above the exit of the infraorbital nerve (Fig. 3.23i, j) and followed by dissection of the periosteum from the orbital floor (Fig. 3.23k, l).




Fig. 3.23
Preseptal transconjunctival approach with transection of the lateral palpebral ligament (the initial stages): (a, b) Anemization and horizontal incision of the lateral palpebral commissure. (c, d) Transection of the inferior crus of the lateral palpebral ligament. (e, f) Dissection of the conjunctiva using scissors (e) or electrosurgery (f). The lower eyelid is pulled away with Desmarres lid retractors; a Jaeger lid plate is used to protect the eyeball. (g) Separation of the lower eyelid retractor (shown with an arrow). (h) Isolation and transection of the tarso-orbital fascia (shown with an arrow). (i, j) Dissection of the periosteum of the infraorbital rim (shown with arrows). (k, l) Periosteum of the orbital floor
The preseptal transconjunctival approach is preferred over the retroseptal approach, since the former approach provides minimal damage to the connective tissue network of the orbit, provides good visualization, and has an insignificant complication rate [12, 158–160].
The main advantage of the transconjunctival approach, in particular when combined with lateral canthotomy, is the absence of cutaneous scars and access to the infraorbital and lateral orbital edges, the lower portion of the medial orbital wall, the upper portion of the anterior wall of the maxillary sinus, the infraorbital nerve, and the medial half of the zygomatic bone [161]. The complication rate is lower than that for the subciliary approach [46, 149, 162, 163], particularly in young patients [12]. The drawbacks of this approach include transection of the lower eyelid retractor and persistent chemosis of bulbar conjunctiva12. Furthermore, a surgeon needs to perfectly know the lower eyelid anatomy; otherwise, there is a risk of “getting lost” in eyelid layers [156].
Endoscopic Approaches to the Orbital Floor
The main drawback of the transconjunctival and subciliary approaches to the orbital floor is difficulty visualizing the posterior edge of a fracture due to its distance (remoteness) and prolapse of adipose tissue. The elevation of the orbital floor toward the orbital apex by 15° is an additional impeding factor. Transantral or transnasal endoscopic approaches are indispensable in these cases [74, 164–169]. Endoscopy provides good illumination and visualization of the fracture for all surgery participants. It allows one to evaluate the completeness of release of the entrapped orbital tissues and the position of the posterior edge of the implant. This approach also makes it possible to trace the course of the infraorbital nerve to avoid its damage13 [170–174]. The procedure is indispensable in cases when a fracture extends to the posterior wall of the maxillary sinus because it allows for better securing of the implant and its distal edge on a small bony spur, orbital process of the palatine bone (Fig. 3.24a–c).


Fig. 3.24
Indications for endoscopic approaches: (a) Extensive posterior fracture with its distal margin confined to the small orbital process of the palatine bone. A CT scan showing the typical length of the fracture is shown for the sake of comparison. (b, c) A typical complication accompanying the attempt to place the distal margin of the implant on the posterior margin of the fracture under insufficient visibility conditions: entrapment of the muscle that used to be released (b) or the orbital fat (c) by the posterior margin of the implant. (d) Transantral approach (an arrow shows the foramen in the anterior wall of the maxillary sinus for placing an endoscope)
Video-assisted endoscopic surgery can be performed even shortly after trauma in patients with persistent palpebral edema which would impede subciliary and transcutaneous approaches [174, 175].
The endoscopic procedures, compared to the transconjunctival approach, in terms of adequate recovery of the initial orbital volume has been shown in cadaver experiments [176, 177] and appreciably numerous clinical studies to be very effective [168, 178–181].
The Transantral Approach
A 4-cm-long incision along the gingivobuccal fold is made to expose the anterior wall of the maxillary sinus. An aperture with the area of 1–1.5 cm2 is formed (Fig. 3.24d); a 4-mm endoscope is introduced into the sinus through the aperture to evaluate fracture length and configuration. While holding the endoscope with his/her left hand and using his/her right hand to hold the instruments, the surgeon carefully removes bony structures entrapping the orbital tissues until the negative traction test is obtained. A rolled flexible implant is placed through the surgical aperture and the orbital floor defect. After being placed in the orbit, the plate is deployed, rotated, and placed over the anterior, medial, and lateral margins of the fracture. If the fracture margins are unstable, the implant is fixed with a screw on the side of the sinus; the orbital floor is supported with an antral balloon, such as a Foley catheter, for 10–14 days [123, 164, 175, 182–184].
The transnasal approach is performed through the extended maxillary sinus ostium while adhering to the same strategy as transantral approach.
It is reasonable to use a combination of subciliary or transconjunctival incisions to assist endonasal or transantral endoscopic approaches to manage extensive posterior fractures of the orbital floor over 4 cm2 in size and to correct long-term enophthalmos after trauma [5, 123, 163, 173, 184–189]. The transpalpebral approach is used to place an implant, while the endoscopic approach is used to visualize the rear margin of the fracture [171].
Although developed rather recently, the endoscopic methods are being continuously improved and are now used as alternative approaches to the fractured area as they provide good visualization and complete anatomic recovery of the orbital floor and eliminate improper position of the lower eyelid in postoperative period [20, 74, 165, 169, 190].
However, endoscopic methods require specific equipment and skills in video-assisted endoscopic surgery; they should be used only by experienced surgeons who know the orbital anatomy well and who are proficient in the conventional methods of orbital reconstructive surgery [172, 191, 192]. The share of endoscopic interventions in a level 1 American regional trauma center is less than 20 % and is confined to managing fractures of the orbital floor, the anterior wall of the frontal sinus, and the zygomatic arch [193]. Based on the survey of 400 American maxillofacial surgeons, Barone and Gigantelli [194] found that only 21.3 % of the respondents use endoscopic methods to manage facial fractures. These were mostly experienced surgeons engaged in private practice. The lack of access to specialized instruments was cited as the main limiting factor.
The two main disadvantages of the endoscopic method are the need for providing temporary antral support to bone fragments and the need for removing a balloon 2 weeks later which is associated with the risk of recurrent prolapse of the orbital floor. The alternative of maxillary sinus tamponade with a gauze sponge is a less suitable method because it is often complicated by orbital cellulitis, hematoma, and persistent diplopia in the postoperative period.
3.3.5 Subsequent Surgery Steps
3.3.5.1 The Release of the Entrapped Tissues and Closing the Bone Defect
Periosteum of the orbital floor is separated along the entire depth of the fracture (Fig. 3.25a). The prolapsed soft tissues are returned to the orbit with a spatula placed in the bone defect zone (Fig. 3.25b, c). When performing this step, it is extremely important to identify the infraorbital nerve as promptly as possible (Fig. 3.25d) to avoid damaging it [195]. Furthermore, it is important to avoid bringing the maxillary sinus mucous membrane into the orbit as it may cause cyst development around the implant. Finally, one needs to avoid excessive pressure exerted on the eye and the optic nerve.




Fig. 3.25
The subsequent steps of surgery orbital floor reconstruction: (a–c) Separation of periosteum along the entire fracture depth (shown with an arrow). (d) The infraorbital nerve (shown with an arrow). (e) Vertical traction test. (f) Forming an implant (“Ecoflon” e-PTFE plate used as an example). (g, h) A straight raspatory is used as a guide to place the implant on the posterior margin of the fracture. (i) The plate is fixed with Beyer incisure (shown with an arrow). (j) Closing the periosteum. (k) Continuous suture of the conjunctiva. (l) Suture of the lateral canthus
The completeness of releasing entrapped tissues is controlled using the traction test (Fig. 3.25e).
The next surgical decision is the choice of an implant14 that would overlap the bone defect by 2–3 mm in all directions (Fig. 3.25f). Plates with minimal (0.5–1 mm) thickness are used in patients without vertical dystopia [196]. If a patient has hypoglobus, the thickness of the implant is equal to the degree of eyeball depression.
The foil packaging of Vicryl suture can be employed to determine the fracture size and contour. The foil sheet is placed into the orbit and pressed against the fractured area. The indent of the bone defect is obtained [93]. After the excess foil around the indent is cut off with scissors, the resulting template is placed on the plate and outlined. Then the plate is used to form an implant. Sometimes it is reasonable to make the implant U shaped by cutting a fragment off its rear edge to prevent infraorbital nerve compression [195].
When closing extensive fractures, one should bear in mind that the rear portions of the orbital floor are angled upward. It is reasonable to use a simple procedure to prevent the rear edge of the implant placed in the orbit from resting in the maxillary sinus. A straight raspatory elevator is placed in the sinus until it reaches the posterior wall of the sinus and is subsequently moved upward until it reaches a bony spur (Fig. 3.25g, h). The raspatory acts as a guide helping the surgeon to achieve proper position of the rear edge of the plate [12]. A repeated traction test is conducted after the orbital implant is placed. If necessary, the plate is fixed on the anterior margin of the fracture with Beyer incisures (Fig. 3.25i).
The final stage of intervention during the subciliary approach includes thorough layered closure of the periosteum (Fig. 3.25j), tarso-orbital fascia, orbicularis oculi muscle, and skin, which prevents implant migration. To prevent postoperative lower eyelid retraction, the length of the tarso-orbital fascia needs to remain unchanged during closure.
Closure of the transconjunctival approach does not require mandatory suturing of the conjunctiva (Fig. 3.25k); this fact does not increase the risk of infectious complications and implant migration or rejection [149, 160]. However, the reconstruction of the lateral ligament and the canthus should be performed very accurately (Fig. 3.25l).
Postoperative treatment includes short-term bed rest (5–6 h), a head-elevated position, a cold pack applied to the orbital zone, and if indicated, analgesic and antiemetic drugs. There is no need in using a compressive bandage; however, if a bandage was used, it must be removed the day after surgery or even earlier in case the patient complains of undue pain. Traction sutures of the lower eyelid margin can be left for several days to prevent its cicatricial contraction. The duration of inpatient postoperative treatment usually depends on the patient’s overall condition and typically is 4–10 days [197].
After discharge, the patients should avoid blowing their nose for at least 2 weeks [198]. Physical activity should be eliminated for a longer period, in particular for people engaged in physically demanding jobs. The physical activity restrictions for patients with blow-out fractures usually lasts for 6 weeks based on the general concept of wound healing and the rate of osteogenesis in patients with orbital fractures [199]. However, Gilliland et al. [200] used an experimental model to find that that as soon as 3 weeks after osteoplasty, the orbital floor which was covered with an implant had the same mechanical strength as that of the intact orbital floor.


The question whether antibiotic treatment is required in patients with blow-out fractures of the orbital floor needs special consideration. There have not been any standardized regimens of antibiotics for this category of patients that have been described in the literature [127, 161, 201].
There is only one reference that showed the use of broad-spectrum antibiotics such as co-amoxiclav or clindamycin to be effective in controlling postoperative infections [202]. Since there is no generally accepted opinion regarding antibiotic use for this problem, Westfall and Shore [161] proposed to use the general surgery standards for prescribing antibiotics depending on wound type:
Type I: clean wound; risk of bacterial infection is less than 1.5 %. The effectiveness and need for preventive antibiotic therapy have not been proved.
Type II: clean-contaminated wound contacting with the upper respiratory tract without massive bacterial contamination. The risk of bacterial complications is 7.7 %; preventive antibiotic therapy is recommended.
Type III: contaminated wound connected with the gastrointestinal tract. The risk of complications is as high as 15.2 %; preventive antibiotic therapy is recommended.
Type IV: infected wound (an old injury, underlying infection, presence of purulent discharge, devitalized tissues or foreign bodies). The risk of wound infection is 40 %; antibiotic therapy is recommended both as a preventive and therapeutic measure.
The presence of a graft or a foreign body in the wound, which is the case if the orbital floor is reconstructed with a graft, significantly increases the risk of infection and is an indication for preventive antibiotic therapy.
The classification of the blow-out fracture wound depends of the affected anatomy. Paranasal sinuses are considered to be sterile. Hence, a blow-out fracture communicating with an intact sinus can be regarded as a clean wound (type I). If a fracture developed in a patient with sinusitis, the wound would then be considered as infected (type IV). The nasopharynx is not considered to be sterile; therefore, a fracture communicating with the nasopharynx should be classified as a clean-contaminated wound (type II). Thus, the blow-out fracture can be classified as any of the four types of surgical wounds (except for type III). Antibacterial treatment is often required immediately after trauma and is mandatory after a surgery using an implant.
Antibiotic therapy should, ideally, be started within the first 3 h after an injury; however, this is often infeasible. Intravenous intraoperative antibiotic therapy started at the time of anesthetic induction very effectively prevents purulent complications [203]. If surgery lasts more than 4 h, a second dose of the drug is given.
The choice of antibiotic agent, duration, and route of administration are extremely important. The absence of past medical history of sinusitis and contact with the oropharynx allows one to use an intravenous infusion of a first-generation cephalosporin (cefazolin).
Third-generation cephalosporins are recommended in all other cases. If there is a risk of saliva contacting the fractured area (i.e., for zygomatic orbital fractures), the recommended drugs include aminoglycosides, amoxicillin, or clindamycin. 2 g of amoxicillin or 600 mg of clindamycin is to be given intravenously during the surgery. 1 g of amoxicillin or (if a patient is allergic to penicillin) 600 mg of clindamycin is continued intravenously for the first 2 days after surgery followed by i.v. infusion of 600 and 300 mg of the drug three times per day, respectively, for 5 days [202].
It is reasonable to include glucocorticoids in the treatment of orbital fractures, since these drugs accelerate regression of orbital edema and the diplopia caused by it without slowing down osteogenesis [93, 203]. Also, posttraumatic enophthalmos can be visualized much earlier and help with the decision whether further surgery is indicated [204].
Injection of 250 mg of methylprednisolone (20-mg dexamethasone) prior to intervention followed by i.v. infusion of the drug three times per day in the same dose (or the dose reduced twice for dexamethasone) every 6–8 h is recommended [12, 205, 206].


Final assessment of surgical outcomes in terms of such criteria as ocular motility and eyeball position in the orbit and presence or absence of diplopia is performed at least 6 months after the repair [75, 76]. In order to avoid additional radiation exposure of a patient, CT scanning should not be performed if an obvious clinical improvement is present.
Proper position of the eyeball in the orbit and the absence of diplopia are considered to be the fundamental indicators of long-term success.
3.3.6 Characteristics of Different Graft Materials
3.3.6.1 Autografts
A number of autograft materials can be used in orbital wall reconstruction (Fig. 3.26) [207, 208]. Full-thickness or split-thickness grafts of the membranous bone of the cranial vault are used most frequently [209, 210], since they are less susceptible to lysis and better retain their initial shape and volume [211, 212]. However, these grafts fail to take the shape of the orbit and therefore are often displaced and need to be fixed to the infraorbital margin [213].


Fig. 3.26
Autografts used for closing orbital wall defects: (a) Cranial vault bones. (b) Internal plate of the iliac anterior crest bone. (c) Conchal cartilage (tissue harvesting site is shown with dashed line). (d) Bony portion of a rib (the cartilaginous portion of the ribs is hatched). (e) Mandible
The widely used graft structures include the internal plate of the anterior iliac crest bone [213–216], a fragment of the bony portion of the rib [217], or a fragment of patient’s mandible [218, 219]. In order to achieve the required congruence with the orbital floor profile, a 2–3-mm-thick fragment of the external layer of compact osseous tissue is harvested from the chin region, behind the homonymous foramen, near the mandibular arch [219], or the mandibular symphysis [220].
The authors believe that the advantages of this method for closing the orbital floor bony defects are as follows: simplicity of harvesting graft material, simplicity of subsequent graft shaping, appropriate size and curvature of the bone plate, the absence of functional disorders when breathing or walking that often occur when the bony portion of the rib or iliac bone are harvested, and the absence of scars or other cosmetic defects at the graft harvest site.
Many different graft sites have been proposed when only a small thin flexible graft is needed. Anderson and Poole [69] used the patient’s periosteal flap; Constantian [221] and Castellani et al. [222] used the conchal cartilage graft (Fig. 3.26c); Johnson and Raftopoulos [223] and Ozyazgan et al. [224] used the cartilaginous portion of a rib (Fig. 3.26d); and M. Kraus et al. [132, 225] and Talesh et al. [226] used the nasal septal cartilage.
A fragment of the anterior wall of the ipsi- or contralateral maxillary sinus can also be used to close small (up to 2 cm in size) orbital floor defects [185, 212, 227–229]; it is implanted into the orbit via the transantral approach using an endoscope [183]. The advantages of the procedure proposed by Kaye [230] include graft harvesting in close proximity to the graft site and the possibility of single-step maxillary sinus cleansing and thorough transantral repositioning of bone fragments in patients with extensive fractures. Other advantages include the absence of cutaneous scars and no risk of perforating the pleura and the dura mater that may occur when harvesting a rib or a cranial vault bone.
A literature review revealed that autografts are still used rather commonly, in particular by neurosurgeons15 [207, 208, 232]. An obvious advantage of bone autografts is that they stimulate osteoconduction, osteoinduction, osteogenesis, and revascularization [213, 233]. Furthermore, autologous tissues are favorably characterized by biocompatibility and the minimal risk of graft infection, migration, or rejection [234]. Hence, this type of graft is primarily used to treat extensive orbital floor fractures when there is a risk of infection at the surgical site [211, 235, 236].
Significant drawbacks of autografting include increased surgical time, additional surgical trauma, graft harvesting complications16, and lysis of one-third of the transplanted autologous tissue resulting in long-term development of enophthalmos, and difficulty associated with forming small grafts [211, 213, 238, 239]. The complexity of graft shaping makes correction of prolapse of the posterior retrobulbar portions of the orbit difficult, while the anterior portions of the reconstructed orbit sometimes turn out to be noticeably smaller than those in the contralateral healthy orbit [240]. As a result, the autologous bone graft does not always adequately substitute for the orbital volume that has increased after the fracture, and therefore does not lead to a high-precision anatomical reconstruction.
3.3.6.2 Allografts
It is more reasonable to use donor tissues, decalcified bone [69, 93, 241], and cartilage [242]. These materials are characterized by good tolerability and simplicity of shaping. Decalcified bone stimulates chemotaxis in the fracture area and transformation of mesenchymal cells to chondroblasts followed by ossification [93, 243].
The cartilage can be located either sub- or supraperiosteally in the orbital adipose tissue fat. A serious drawback of cartilaginous grafts having no epichondrium17 is that they undergo gradual resorption within 1–1.5 years. This reabsorption has been confirmed by CT data. Hence, when using cartilaginous tissue, one needs to achieve intraoperative overcorrection of the enophthalmos by 1.5–3 mm. However, this may be associated with the risk of developing hypertopia of the eyeball.
Closing bone defects using a composite consisting of lyophilized cartilage and heterogeneous (bovine) bone morphogenetic protein is more reasonable alternative to solving the problem of cartilage graft resorption. Addition of protein inducing osteogenesis significantly accelerates the slow process of calcification/ossification of the donor cartilage tissue, and it overcomes the process of cartilage tissue resorption [244]. Osteogenetic activity of recombinant bone morphogenetic protein and fibroblast growth factor has been confirmed experimentally [245, 246].
The dura mater [209, 247–249] and thigh fascia lata [250, 251] are used for orbital floor reconstruction in patients with small fractures up to 2 cm2; these grafts can be easily shaped and implanted into the orbit. However, the use of these materials is limited, since balloon support on the side of the maxillary sinus is required when using these tissues to close a larger orbital floor defect.
The use of decalcified bone, cartilage, and the dura mater as donor tissue has declined significantly primarily due to the increasing risk of transmitting causative agents of numerous diseases with the graft. Thus, although the dura mater is one of the main osteoplastic materials in Europe, it has not been used in the United States because of the risk of contaminating a recipient with prions, the causative agents of Creutzfeldt–Jakob disease [209].
The cost of using allografts is also considerable because of the necessity for the establishment of tissue banks to conduct bacteriological and virological testing of donor material following of the rules for its preservation and storage [241]. In this regard, synthetic materials have considerable merit over donor tissues.
3.3.6.3 Exgrafts
Nonbiological materials such as resorbable and nonresorbable solid and porous polymers as well as 0.3–1.0-mm-thick titanium mesh constructs are most commonly used for surgical management of orbital floor fractures [7, 46, 87, 232, 252–254]. The choice of a material for closing bone defects primarily depends on the area of defect.
Resorbable polymer grafts are the main material to close small bone defects up to 2 × 2 cm in size without evident enophthalmos and hypoglobus [231, 255, 256]. A linear-type trapdoor fracture that can occur in children is a typical example of such an injury. Films “Gelfilm” [75, 257–259], “Seprafilm”18 [260], polydioxanone19 [118, 158, 209, 238, 255, 261], and Vicryl20 [262] are used in these cases. Review of published data shows that the two latter materials are used most commonly.
Polydioxanone (PDS) has recently been widely used in clinical practice [231, 263]. An implant made of 0.15-mm-thick perforated polydioxanone foil less than 20 mm in diameter is not inferior to a 0.3-mm-thick titanium mesh in terms of its mechanical strength [264]. Hence, PDS is used to close bone defects up to 2 cm2 in size [265].
The attempts to use 0.25- and 0.5-mm-thick plates to manage larger fractures were unsuccessful because intense PDS resorption started after 2–3 months and the implant lost its mechanical strength. The newly formed connective and osseous tissues that replaced the biodestructed PDS [265] fail to perform the tectonic function even in medium-length fractures because they bulge into the maxillary sinus and cause late enophthalmos [266]. As a result, if these agents were used to repair a large fracture, one needs to overcorrect and that will lead to inevitable diplopia in the early postoperative period [265].
Other complications of using PDS are the development of diplopia and exophthalmos which seems to be caused by pronounced response of tissue to the material [255], plate displacement [267], and severe cicatrization in the implantation zone. MRI can often show the formation of liquid- and gas-containing cavities [268].
Closure of a small orbital floor defect up to 2 cm2 with an ETHISORB Dura Patch21 is associated with a much smaller rate of diplopia and enophthalmos [255, 261].
The serially produced 4-mm-thick Vicryl plate consists of 24 layers; thus, it can be separated into thinner implants that can be easily shaped and do not need to be fixed in the orbit. Vicryl is characterized by unique physical/mechanical properties that prevent compression of the optic nerve, lacrimal sac, or the extraocular muscles. The material is well tolerated by orbital tissues, bones, and the mucous membrane of paranasal sinuses and does not impede osteogenesis [269]. However, this material can cause an inflammatory response of the lower eyelid tissues in 14 % of cases, which may lead to cicatrization of the lower eyelid [270]. Furthermore, polyglactin should not be used to close large orbital floor defects and to perform contour reconstruction of the orbit because it starts to lose its original strength as soon as 1 week after implantation. Only traces are observable 1 month later and 4 month after surgery Vicryl is completely resorbed.
Caution is needed in choosing a mesh made of polyglycolic acid–polylactic acid copolymer (LactoSorb)22 to close extensive orbital fractures [271], since the inevitable hydrolytic destruction of the implant causes enophthalmos. In addition, the mandatory rigid fixation of the plate to the infraorbital margin is associated with the risk of developing local inflammatory response that forces one to remove the implant at a later date [272]. The next generations of these implants (Resorb X(®), SonicWeld Rx-System®) and the composite consisting of polylactide and hydroxyapatite may be more applicable.
Lactic acid homopolymers with resorption duration ranging from 1 to 5 years are promising osteoplastic materials [273–277]. Despite their small thickness, polylactide implants with added trimethylene carbonate exhibit sufficient mechanical strength, can be easily shaped when heated to 55 °C to duplicate the orbital profile, and are also biocompatible. The resorbable properties of the materials do not require reoperation to remove them [271]. The above listed properties of polylactide make favorable comparison with the nonresorbable implants HAp and coral. The latter implants, HAp and coral, have a disadvantage because they need to have an increased thickness due to their fragility. They also have a rough surface, and the implant shape and curvature are determined by the manufacturer and cannot be changed. Finally, there is a need for special equipment for titanium constructs as well.
Experiments with using polylactide to close extensive bone defects showed that it is biocompatible with insignificant inflammation and capsule formation around the implant and osteogenesis in the bone defect area after 9 months. However, the implant lost its initial mechanical strength after 16 weeks, and by the end of the experiment, 40 % of plates were completely resorbed. The rest of the implants were severely deformed because of encapsulation and osteogenesis occurring in the adjacent areas [278]. Threefold thickening of the material 1–1.5 years after it was placed in the orbital tissues is another disadvantage [279]. Furthermore, because polylactide is radiologically transparent, CT monitoring of the implant position in the orbital floor forces one to use more expensive MRI for monitoring [274, 275, 279, 280]. There is an additional drawback in that the polylactide implants are very expensive.
1.5-mm-thick screws intended for fixing serially manufactured Inion polylactide plates turned out to be extremely fragile, and the 2.5-mm-thick ones are too thick (Fig. 3.27). In addition, their biodestruction by-products cause significant tissue response in the implantation zone which limits the number of screws that can be used during a surgery.


Fig. 3.27
Polylactide implants (using products manufactured by Swiss company “Synthes” as an example): (a, b) The amorphous ultrastructure of the copolymer based on d-lactide and DL-lactide monomers. The material undergoes hydrolytic destruction whose rate depends on copolymer composition. (c) Absorbable miniplates and screws
Thus, because polylactide has so many negative attributes, and there is no comprehensive data that shows it is better than titanium implants, it will not be used as the main material for closing extensive orbital floor defects in the near future [281]. However, the use of polylactide and polyglycolic acid meshes, plates, and screws may show promise for orthognathic surgery and pediatric cases. The slow hydrolytic destruction of the plate which occurs over several months allows for unimpeded growth of facial and cranial bones, whereas metal constructs would decelerate this process and cause facial asymmetry [1, 277, 282, 283].
Solid nonresorbable polymers have been used for over 40 years. They include polymethyl methacrylate (PMMA) [284], polyethylene (PE) [94], and Supramid [75, 285–287].
Solid Teflon has been mentioned as a material that can be used for orbital floor reconstruction [211, 235, 288]; Hardin [289] has performed 500 surgeries using this polymer.
Silicone implants are still used rather commonly [214, 290, 291]. According to Courtney et al. [127], polydimethylsiloxane is used in 66 % of orbital floor reconstruction surgeries performed in Great Britain.
Tercan’s proposition [292] to use steel wire to reinforce a 0.6-mm-thick silicone plate makes it suitable for closing extensive fractures of the orbital floor and facilitates its fixation to the infraorbital margin. Furthermore, the mesh implant is visible on CT scans.
The disadvantages of using silicone, which has a nonporous, solid structure, include the risk of implant migration under the lower eyelid skin, to the nasal cavity or into the maxillary sinus [293, 294]. Another serious complication of using silicone is development of chronic perifocal inflammation impeding osteogenesis in the bone defect area [200, 269] and formation of a pseudocapsule lined with stratified squamous epithelium of the conjunctiva around the silicon. Implant encapsulation may result in formation of a cutaneous or sino-orbital fistula, persistent diplopia, vertical and axial dystopia, or cellulitis [295]. When staying in the orbital floor for a long time, silicone causes bone tissue resorption which may lead to maxillary sinus involvement in the pathological process in up to 70 % of patients.
Twenty-year follow-up of large patient cohorts has shown that silicone implants had to be removed because of complications in 13–14 % of cases [296]. Explantation was performed on average 4.3 years after surgery, although complications can emerge 10, 15, and even 25 years after osteoplasty [293, 297, 298]. Because of the high rate of late complications, many authors prefer using autologous conchal cartilage rather than silicone to close small fractures up to 1.5 cm2 and using autologous bone grafts to substitute in larger defects.
Titanium is another common material for orbital floor reconstruction [240, 299]. The biocompatibility of titanium is attributed to the fact that its atomic number (22) is close to that of calcium (20), the main mineral component of bone [300]. Furthermore, titanium is characterized by the absence of evoked potentials on the surface, which makes it “invisible” for immunocompetent cells and eliminates the risk of metallosis. Unlike steel, titanium is capable of osseointegration; this fact explains the low risk of infection even when titanium is implanted in the oral cavity. Due to rigid fixation to the adjacent bone structures, there is zero probability of migration and rejection of titanium constructs. Furthermore, they ensure more accurate reconstruction of the orbital wall contour compared to bone grafts [240]. However, titanium constructs are believed to impede rapid callus formation, since the rigidly fixed fragments do not undergo compression required for it [277].
The relative simplicity of graft shaping, hypoallergenicity, corrosion resistance, nontoxicity, and non-carcinogenicity have made titanium an osteoplastic material that has been successfully used for the past 40 years [239, 301]. Coating the surface of titanium implants with mesenchymal stem cells which accelerates biointegration seems to be rather promising.
Titanium miniplates (Fig. 3.28a) proposed by Champy are poorly applicable for managing orbital fractures because these implants are difficult to be properly shaped and their linear size is inconsistent with the thin orbital walls. Furthermore, miniplates placed on the orbital margins increase sensitivity to cold, are easily palpable, and can deform the periorbital contour in patients with thin skin, which is the reason for explantation in 5–6 % of patients23 [277, 303–306].


Fig. 3.28
Titanium implants for orbital reconstruction: (a) Miniplates. (b) Modern modifications of screws for fixing mini- and microplates whose use does not require drilling. (c, d) Titanium plate (c) and mesh (d) for closing extensive bone defects. (e) Titanium orbital implant manufactured by Synthes company (Switzerland). Due to its small thickness (0.2–0.4 mm) and numerous preformed cuts, the plate can be easily shaped. Three bulges rigidly attach the implant to the infraorbital margin. (f) A 3D CT image of the plate
The drawbacks of miniplates have stimulated design of microplates as thin as 0.4–0.6 mm. They cannot be palpated under the skin, do not deform the orbital contours, and securely fix small fragments. Miniplates however cannot immobilize these small fragments due to screw diameter of 1.2–1.3 mm and the distance between the holes of 4 mm. Unfortunately, when implanted onto the infraorbital margin, microplates cannot resist cicatricial contraction of soft tissues in the zygomatic area [307].
Because it is extremely difficult to provide rigid fixation of laminar grafts for fractures of 2–4 orbital walls (Fig. 3.28c–g), these are the main indication for using titanium mesh. In these cases, titanium acts as a platform to host the grafts [308]. A significant drawback of the mesh is that it is very difficult to implant it because of sharp edges that hook soft tissues (Fig. 3.28e). Mesh explantation is also a challenging procedure as the mesh becomes interwoven with cicatricial tissue [12].
The attempts to implement vitallium (the cobalt–chromium–molybdenum alloy) in clinical practice failed as this material has no benefits compared to titanium [309]. Tantalum is not used, as its strength is lower than that of titanium.
Silicon nitride has shown biocompatibility and good physical/mechanical properties in experimental studies. As opposed to titanium, it does not generate artifacts during radiological examination and can be attached to bones lined with mucous membrane. Carbon implants are currently undergoing preclinical trials.
Thus, nonresorbable and resorbable nonbiological implants for orbital floor reconstruction are extensively used by surgeons due to their biocompatibility, chemical stability, and commercial availability. Complications observed in clinical use such as implant migration, rejection, recurrent hemorrhage into the subcapsular space, and infection of the material occur because the newly formed connective tissue does not grow into this type of implants [310]. The risk of purulent complications when using solid implants is especially high in patients with traumatic anastomosis with the maxillary sinus [272]. Hence, porous synthetic materials have recently been becoming more common [207, 208].
Salyer and Hall [311], Mercier et al. [312], and Gas et al. [238] have successfully used implants made of aragonite. It is the skeleton of marine reef-building coral belonging to the genus Madrepora which has been subjected to hydrothermal treatment according to the procedure proposed by Roy and Linnehan [313]. The rigidity of the resulting hydroxyapatite (HAp) makes it possible to close even large orbital floor defects (Fig. 3.29). After fragment reposition, hydroxyapatite blocks can also be implanted in the maxillary sinus where they will support the reconstructed orbital floor [314].


Fig. 3.29
Implants made of coral-derived hydroxyapatite: (a) The labyrinth-arch network of interconnected pores 150–500 μm in diameter, which resembles the haversian system (b) of human compact bone and provides rapid tissue colonization of hydrophilic coral. (c, d) Biocoral coral-derived osteoplastic implants (manufactured by Inoteb)
Secure fixation of the coral-derived HAp to the underlying bone is observed as early as 3 months after implantation. Tissue colonization ends 4 months after osteoplasty; however, the newly formed osseous and connective tissues occupy less than 20–30 % of the porous space volume of HAp [315]. As a result, an implant staying in the tissues for over a year is partially resorbed via hydrolytic destruction [315–319]. This presumably accounts for the frequent development of enophthalmos in the long-term period after implantation using HAp [320]. For example, the total rate of complications accompanying bone defect closure using Biocoral hydroxyapatite was found to be 9.4 % [320].
To process coral, the operating room needs to be equipped with a diamond drill burr. After shaping, dust needs to be removed from the plate using normal saline solution and a brush, which causes a certain inconvenience during the surgery. The attempts of rigidly fixing HAp with wire or screws fail because of the fragility of the material. The use of coral for facial areas with thin layer of superficial soft tissues is also a challenge. Thus, the difficulties associated with shaping, fixating, and tissue coverage are responsible for the fact that coral-derived HAp remains an auxiliary osteoplastic material that can be used only in some situations, which are mainly for substituting large defects.
Less expensive implants made of synthetic HAp are also used for orbital wall repair [321]; however, they are characterized by even higher fragility.
Cement based on calcium phosphate β-Ca3[PO4]2 with pore size of 100–300 μm and porosity of 36 % is a promising material for reconstruction of damaged orbital walls [322]. Strength of this material is 2.5-fold higher than that of coral [323]. It was found in an experiment involving rabbits that a ceramic implant is resorbed within several months and is replaced by newly formed compact bone [324]. Osteoinductive properties of β-Ca3[PO4]2 can be enhanced by passivating its surface with recombinant bone morphogenetic protein [325]. The material is already being used in neurosurgery to separate the cranial cavity and accessory sinuses of the nose where it has demonstrated biocompatibility and ability of epithelialization [323].
Hoffmann et al. [326] used implants made of Bioverit, the nonresorbable porous glass ionomer cement with the formula SiO2–Al2O3–MgO–Na2O–K2O, for orbital reconstruction. Klein and Glatzer [327] have reported in a small series the use of individual Bioverit II bioceramic implants to correct enophthalmos. They found that a high-speed drill needs to be used for cement shaping. The implant needs to be at least 3 mm thick for the planned use of titanium screws. Furthermore, the plate needs to be placed subperiosteally.
The Neuro-Patch dura mater prosthesis made of microporous nonwoven aliphatic polyester urethane can be used to close small orbital floor defects up to 1 cm2 in size [328].
However, porous polyethylene implants manufactured by Porex Inc. (United States) and Synthes (Switzerland) (Fig. 3.30) are now most commonly used [35, 172, 178, 197, 329].


Fig. 3.30
Implants made of Medpor porous polyethylene (manufactured by Porex) and Synpor porous polyethylene (manufactured by Synthes): (a) The pore space, which is a system of unordered pores 150–500 μm in diameter occupying ~50 % of the implant volume. (b) Rough surface of porous PE. (c) Tunnel implants for closing extensive orbital floor defects. (d–f) Polyethylene plates reinforced with a titanium mesh
High biocompatibility and porous structure of PE provides rapid fusion of the implant and the adjacent tissues [310] provided that the implantation site is well vascularized [330]. Dougherty and Wellisz [331] examined a model of zygomatic orbital fracture and found rapid epithelialization within 1 week and intergrowth of PE with fibrovascular tissue, as well as signs of osteogenesis inside the implant as soon as 3 weeks after the surgery. Reliable fusion with the adjacent anatomical structures was confirmed by subsequent clinical and morphological findings [332].
Since the volume of PE staying in the orbit for a long time is constant, there is no need to overcorrect during surgery. The risk of infection is significantly reduced due to the possibility of saturating the implant in an antibiotic solution and tissue colonization of polyethylene [202]. As a result, the rate of complications for using PE as an orbital implant is no higher than 5.5–6 % [320, 333].
0.85- and 1.5-mm-thick preforms are characterized by elasticity and can be easily processed using a scalpel and scissors [334, 335]. A 3-mm-thick plate can also be processed but needs to be preheated in hot water.
It can be rather difficult to achieve a stable position of the conventional implant model in patients with fractures of the posterior portion of the orbital floor or its extensive defects larger than 2 cm2. Su and Harris [336] used 2–3 polyethylene plates placed in a shingle-like manner without any fixation to close extensive inferomedial fractures. Furthermore, modified laminar implants having internal canals, which allows one to reliably fix them using mini- and microplates, are used to treat this complex class of fractures [337] (Fig. 3.30c). Titanium-reinforced polyethylene implants are the most recent successful development for closing this type of fracture [338–340] (Fig. 3.30d–f).
The drawbacks of polyethylene include its radiotransparence: this material can be visualized in CT scans only after the vascularization process is finished [202]. It turned out that implantation of PE directly under skin without using proper periosteal or fascial coating is fraught with early and, in particular, late exposure, with its frequency being higher than 10 % [341]. Furthermore, polyethylene fails to duplicate facial contours because of its excessive rigidity [330].
Implants made of various configurations of polytetrafluoroethylene (PTFE)—nonporous films and porous plates—have recently been extensively used in craniofacial surgery. The modern applications of PTFE include facial contouring surgery, suspension surgeries in patients with facial nerve paralysis, and malar-, mento-, and rhinoplasty [342, 343].
Elasticity, ease of shaping, chemical and biological inertness, availability, and inexpensiveness of PTFE make it a promising material for closing the orbital floor defect [344]. PTFE film may be used to close small bone defects up to 1.5 cm. Furuta et al. [345] used PRECLUDE polytetrafluoroethylene dura mater substitute (manufactured by Gore & Ass. company) to compensate for periosteal deficits. Ma et al. [346] successfully used 2-mm-thick plates made of Proplast I, a composite material consisting of the mixture of PTFE and carbon fibers to close a blow-out fracture.
Six-month experiments involving repair of bone defects with Ecoflon porous polytetrafluoroethylene implants manufactured in Russia have demonstrated stability of the implant position, minimal phagocytic response (Fig. 3.31a) and delicate capsule formation around the polymer (Fig. 3.31b) and ingrowth of newly formed connective (Fig. 3.31c) and osseous (Fig. 3.31d, e) tissue into its pore space. This occurred in some areas even with hematopoietic bone marrow (Fig. 3.31f) (Astakhov and Nikolaenko 1999–2005).


Fig. 3.31
Tissue responses accompanying implantation of Ecoflon porous PTFE manufactured in Russia into the orbit. Hematoxylin and eosin staining: (a) The absence of macrophagal response 1 week after surgery, ×100. (b) Encapsulation of the implant 2 weeks after the experiment was started, ×100. (c) Mature connective tissue inside PTFE 1 month after implantation, ×125. (d, e) Osteoblast proliferation in PTFE micropores (d) giving rise to an islet of newly formed osseous tissue (e) 6 months after surgery, ×200. (f) Hematopoietic bone marrow in the newly formed bone tissue (6 months after implantation of PTFE)
The 8-year experience of using PTFE in clinical practice demonstrated that a PTFE plate can be easily shaped using scissors and a scalpel due to physicochemical properties of this porous material (Fig. 3.32a–d). Elasticity of the polymer allows the polymer to duplicate all the curvatures of the S-shaped profile of the orbital floor (Fig. 3.32e). Rough surface provides certain adhesion to the adjacent tissues and eliminates the need for rigid fixation of the implant to the infraorbital margin. Formation of clear images on CT slices allowing one to easily control the insertion position is an obvious advantage of the polymer (Fig. 3.32e, f).


Fig. 3.32
Physical/mechanical properties of Ecoflon porous polytetrafluoroethylene (manufactured in Russia): (a) Elasticity and capability of reversible deformation. (b, c) Shaping using scissors and a scalpel. (d) Possibility of applying sutures using surgical needles. (e) Plate location on the orbital floor. (f) PTFE is clearly visualized on CT scans
Thus, high biocompatibility, no risk of infection transmission, approved manufacture, and acceptable costs gradually make porous polymers the main material for orbital floor reconstruction.
3.3.6.4 Xenografts
Cheung et al. [347] reported the first experience of using Permacol porcine dermal collagen xenograft to reconstruct the orbital floor. No complications were observed during the surgery and in early postoperative period. However, there was a late onset of hypertopia and restriction of infraduction.
Implant removal did not significantly improve the condition of the orbital tissues. Gross scarring of the inferior rectus muscle was detected during a repeated orbitotomy. Histological examination showed an inflammatory response with pronounced giant cell reaction. Thus, despite such advantages of the xenograft as mechanical strength and easy processing, it is unreasonable to use for managing orbital fractures. Better results will probably be achieved when using bovine and porcine pericardium, hydroxyapatite carbonate derived from porcine compact bone tissue [348], Surgisis ES lyophilized acellular matrix of submucosal tissue of porcine intestine [349], or Bio-Oss bovine bone matrix for this purpose.

Summarizing all the facts mentioned above, we would like to draw a conclusion that early and thorough single-stage treatment needs to be used to manage blow-out fractures. The implant used needs to fulfill a number of requirements, including:
1.
Simplicity of the shaping and subsequent implantation procedures
2.
Ability of the implant to support the orbital structures
3.
Stability of the initial position due to rapid integration with the surrounding tissues
4.
Resistance to bacterial contamination
5.
Clear visualization of the implant on CT or MRI imaging
Modern nonbiological porous materials such as porous polyethylene, coral-derived hydroxyapatite, and porous polytetrafluoroethylene that has been designed by us and is highly competitive with the best international analogues in terms of its chemical and physical/mechanical properties meet these requirements to a great extent.
3.4 Complications of Blow-Out Fractures of the Orbital Floor and Their Surgical Repair
According to Folkestad and Westin [350], more than 80 % of patients have certain sensory or visual disturbances even 5 years subsequent to the trauma. The most conservative estimates show that the complications are associated with the surgical repair in 10–15 % of cases. Potential causes include the approach used, the material selected to close the bone defect, the foreign body reaction to the implant, or the inadequate scope of the surgical repair [237, 351]. Thorough description of the various complications that can develop in patients with blow-out orbital floor fracture is presented below.
3.4.1 Orbital Hematoma
There are five orbital compartments that can potentially accumulate blood: the intraconal, the extraconal, the subperiosteal compartments, the sub-Tenon’s space, and the space below the optic nerve sheaths.
Blunt orbital trauma most typically results in a retrobulbar (intraconal) hematoma that is located within the muscular funnel and is caused by rupture of short posterior ciliary arteries [352] or, less frequently, in a subperiosteal hematoma [353]. The hemorrhage into the orbital cavity can also be delayed [354–356].
The first case of blindness caused by retrobulbar hematoma which developed during repair of an orbital fracture was reported in 1950. The current rate of this complication is 0.3–0.5 % [287, 356].
The main reason behind intraoperative, or early postoperative, hemorrhagic complications is disturbance of the orbital branchlet, which runs from the infraorbital artery 13–17 mm below the orbital margin and anastomoses with the vessels of the inferior rectus and inferior oblique muscles, as well as the lacrimal and dorsal nasal arteries. Sometimes 2–3 orbital branchlets run from the infraorbital artery every 3–4 mm. They can be cauterized without any risk to the orbital circulation. The orbital branchlet can be easily mistaken for the infraorbital artery when the orbital floor is displaced downward and the adipose tissue surrounds the infraorbital neurovascular bundle [357].
In patients with extensive orbital fractures, the effused blood is easily evacuated into the paranasal sinuses and the nasal cavity [356]. In a fracture without fragment displacement, blood remains in a closed space limited by the bones and the tarso-orbital fascia, which risks the development of the orbital compartment syndrome. This risk is higher in young people who have a well-developed network of connective tissue orbital bundles (Figs. 1.9, 1.10, 1.11, 1.12, and 1.13) that retains blood in the retrobulbar orbital compartment.
The orbital portion of the optic nerve is 25 mm in length. This is 7 mm longer that the distance from the posterior pole of the eye to the orbital apex. This extra 7 mm give it an S shape and thus is mobile. This and the mobility of the tarso-orbital fascia can compensate for the increase in intraorbital pressure to a certain extent but cannot protect the optic nerve and the globe against pronounced intraorbital and intraocular hypertension in patients who have a massive hematoma [358].
The rapidly increasing intraorbital pressure causes pronounced pain, diplopia, exophthalmos, periorbial ecchymosis, chemosis of the bulbar conjunctiva, subconjunctival hemorrhage, corneal edema caused by ocular hypertension, optic disk swelling, and external and internal ophthalmoplegia with afferent pupillary defect [358–360]. The raised orbital pressure caused by the bleeding may result in compression of the optic nerve and the central retinal artery which then leads to a risk of irreversible loss of visual acuity up to complete blindness [352, 356, 361, 362]. Since 100–120 min of ischemia cause retinal cell death, early diagnosis and emergency surgical and therapeutic assistance are extremely important [361].
The diagnosis of this condition relies on the following symptoms: sudden onset, pronounced strain in the orbital tissues, acute pain, and abrupt reduction of acuity.
In patients with intraoperative development of hematoma, the main signs include strain in the orbital tissues, an abrupt increase in ocular pressure, and pupillary dilation.
CT is a mandatory diagnostic tool that allows one to evaluate the hematoma location and size, the accompanying injuries to bones and the cerebrum, and the presence of foreign bodies in the eyeball, orbit, or the cranial cavity. When used as an urgent method of examination, CT is superior to MRI as it provides better visualization of bone structures and is more informative in terms of evaluating the condition of soft tissues. It also and does not have the risk of an MRI causing an additional injury by affecting an undiagnosed metallic foreign body [362].
3.4.1.1 The Treatment Algorithm
Patients with suspected retrobulbar hematoma need to undergo an urgent ophthalmological examination, lie with their head elevated, and have an ice pack placed on the orbital area.
Conservative treatment needs to be started immediately (without waiting for CT or ultrasonography results):
Intravenous bolus injection of 8 mg of dexamethasone
Intravenous bolus injection of 80–100 ml of 20 % mannitol solution for 3–5 min followed by a 24-h infusion (2 g mannitol/kg body weight)
Oral administration of 250 mg of acetazolamide twice per day with a 12-h interval
Instillation of 0.5 % timolol solution
In addition, blood pressure needs to be thoroughly controlled, and because the Valsalva maneuver can exacerbate the problem by increasing blood, ocular, and intracranial pressure, it needs to be avoided by eliminating nose blowing and vomiting.
Increased ocular pressure and/or pronounced vision loss (up to no light perception) in addition to the tense orbital tissues and the lack of effect of conservative treatment are an indication for performing emergency orbital decompression using various surgical approaches to prevent compression of the optic nerve and orbital vessels [360, 363]. According to the survey performed among 288 British maxillofacial surgeons, retrobulbar hematoma requires surgical management in 90 % of cases [359]. It should be mentioned that loss of light perception is not a contraindication for the emergent surgery, because even in cases where all light perception has been lost, there can be restoration of central vision if the surgery is done promptly [361, 364].
Lateral canthotomy with inferior cantholysis and orbitotomy are the simplest procedures for orbital decompression.
Lateral canthotomy is performed under local (infiltration) anesthesia (Fig. 3.23a–d). The palpebral commissure is incised up to the lateral orbital margin using straight scissors; the inferior crus of the lateral palpebral ligament is then transected.
Inferolateral orbitotomy is performed if the initial decompression is insufficient. In addition to dissection of the tarso-orbital fascia, one needs to open the 3D network of well-developed 0.5-mm-thick connective tissue membranes around the inferior rectus and oblique muscles, as well as at the level of the eyeball equator [106].
The membranes have a round orientation in the plane where the oblique muscles are attached and are mostly oriented radially at the level of rectus extraocular muscles. Thus, dissection should be performed along connective tissue bundles in order to separate them without disturbing the 3D structure. Taking into account the risk of recurrent orbital hematoma, no sutures are placed on the orbitotomy incision.
Opening of the subperiosteal hematoma assumes that the targeted access to it, both from the orbit and through the adjacent sinus, is used.
3.4.2 Orbital Emphysema
Clinically significant emphysema of the orbit and periorbital tissues, defined as causing disturbances of extraocular muscle function and vision, caused by repeated sneezing or nose blowing is a rather rare24 complication of blow-out orbital floor fractures [12, 366–368]. Rupture of the mucous membrane of the maxillary sinus is a prerequisite of the development of this complication, which is indicated by the fact that emphysema is accompanied by blood in the sinus [369].
In addition to keeping a patient informed about the recommended behavior, it is reasonable to plug the ipsilateral nasal passage, thus preventing air from getting into the orbit [370].
Emergency drainage is recommended for patients with pronounced orbital emphysema (the procedure is thoroughly described in Chap. 4 devoted to fractures of the medial orbital wall).
3.4.3 Infectious Complications
The reasons for development of orbital infection include sinusitis, dental and periodontal pathology, hematogenous dissemination, penetrating orbital injury, and inadequate asepsis and antisepsis during surgery [161, 371–373]. Maxillary sinus tamponade considerably increases the risk of infectious complications although the reasons for that are not clear [235].
Underlying or accompanying sinusitis is responsible for 70–90 % of all orbital infection cases. Therefore, thorough examination of the sinuses is essential when evaluating orbital trauma [374].
Purulent complications are most frequently associated, in descending order, with the ethmoidal labyrinth (75–90 %) or the frontal and maxillary sinuses. Isolated inflammation of the sphenoidal sinus is a very rare condition, and if present, it usually is a complication of ethmoiditis.
The prevalence of ethmoidal labyrinth pathology is caused by insignificant thickness of the medial orbital wall, presence of two ethmoidal foramina (the preformed route), and sometimes additional perforations, which are normal variants [375]. The orbital floor may also have congenital defects or dehiscences, while the fracture caused by trauma allows the infection to directly enter the orbit from the sinus. Valveless facial veins facilitate transmission of microflora from the sinuses.
The microbial population in paranasal sinuses is typically represented by Streptococcus (pneumoniae, agalactiae, equinus), α-hemolytic Streptococcus, less frequently, Haemophilus influenzae and Staphylococcus aureus. The combination of both aerobic and anaerobic bacteria (bacteroides, peptostreptococci, peptostaphylococci, Pseudomonas aeruginosa, and Haemophilus influenzae) as well as rare Gram-negative bacteria, which sometimes are insusceptible to antibiotic therapy, is typical of adult patients [184]. Blood in the sinus accompanying the fracture provides a favorable environment for bacterial growth.
Chronic dental and periodontic pathology is the second most common reason for infectious complications. For example, tooth extraction is the reason for 22.5 % of all purulent inflammations of the maxillary sinus.
Nasopharyngeal infections in high-risk patients (AIDS, diabetes, chronic diarrhea with metabolic acidosis) are the third most common infections. Administration of glucocorticoids, immunosuppressants, and chemotherapy drugs is associated with a high risk of rhinocerebral fungal infection caused by phycomycetes, ascomycetes, and other nasopharyngeal saprophytes. In ~5 % of cases, when sinus aeration is disturbed, inflammatory complications are caused by Aspergillus fungi in combination with other causative agents.
3.4.3.1 Clinical Presentation
The orbital septum plays a crucial role in the presentation, course, and treatment of orbital infections. It is attached to palpebral cartilages and orbital margins and separates the orbit into two compartments, the anterior preseptal and posterior, postseptal. The eyelids and the lacrimal sac are located in the anterior compartment. The eyeball, the optic nerve, the extraocular muscles, neurovascular structures of the orbit, and adipose tissue are located behind the orbital septum. The fascia to a certain extent impedes inflammation spreading from the anterior compartment to the posterior one and vice versa.
There are five clearly defined forms of orbital infection: preseptal cellulitis, postseptal cellulitis, subperiosteal abscess, orbital abscess, and cavernous sinus thrombosis (Fig. 3.33) [376].


Fig. 3.33
Clinical forms of orbital infection (the axial cross-section of the orbit): (a) Postseptal cellulitis. (b) Orbital abscess. (c) Subperiosteal abscess. (d) Cavernous sinus thrombosis
Preseptal (periorbital) cellulitis, in the posttraumatic setting, develops when infection spreads through a bone defect; periorbital swelling results from impeded blood outflow along the superior ophthalmic vein. The clinical examination is limited by palpebral swelling closing the palpebral opening and hyperemia of periorbital skin. The main difference of this pathology from a postseptal process is that it is not characterized by involvement of the patient’s systemic condition, exophthalmos, limited ocular motility, or reduced visual acuity.
Postseptal cellulitis is the acute diffuse purulent inflammation of orbital tissue behind the tarso-orbital fascia. Fortunately it is a rare complication of orbital fractures. Its rate is usually less than 1 %. Recent sphenoethmoiditis (1–2 weeks prior to trauma) or sphenoethmoiditis developed during the early period after trauma (no later than within 5 weeks) is the inciting factor; however, cellulitis cases have also been described in patients without past history of this disease [371]. Forceful nose blowing aggravates the emergence of the pathological process [368].
In most cases, the pathology is unilateral and is characterized by acute onset. The patients complain of general fatigue, orbital pain aggravated by eyelid palpation and eye movements, and diplopia accompanied by fever and the characteristic changes in blood in patients with infections, leukocytosis, shift in band neutrophil count, toxic granulosity of neutrophils, aniso- and poikilocytosis, and elevated ESR. After several hours, the general symptoms of infection are accompanied by hyperemia and marked palpebral swelling, conjunctival chemosis, exophthalmos with partially or completely limited ocular motility, and sudden vision loss caused by compression of the optic nerve secondary to swollen tissues occluding the central retinal artery.
In almost half of cases, postseptal cellulitis causes orbital or subperiosteal abscess formation that requires drainage along with intensive antibiotic therapy.
In addition to the past medical history and the characteristic clinical presentation, diagnosis is facilitated by X-ray imaging of the orbital and paranasal sinuses.
In 10–20 % of cases, the course of orbital cellulitis is aggravated by maxillary osteomyelitis, decreased vision caused by toxic optic neuropathy (3–11 %), superior orbital fissure syndrome, and orbital apex syndrome. Purulent processes in the orbit can be complicated by thrombophlebitis of the veins in the orbit, pterygopalatine plexus, cavernous sinus, and internal jugular vein which subsequently may be followed by development of severe intracranial complications.
Orbital abscess is an encapsulated purulent cavity located in the muscular funnel. For an appreciably large abscess, the clinical presentation resembles that of postseptal cellulitis; thus, differential diagnosis requires performing CT and MRI.
Subperiosteal abscess is characterized by accumulation of pus between the bony orbital wall and the periosteum. In 80 % of cases, it is localized in the superomedial quadrant of the orbit. Its symptoms are marked edema and hyperemia of the upper eyelid, disturbed upper eyelid motility, and eyeball displacement in the direction opposite to the abscess location accompanied by limited ocular motility and exophthalmos. In patients with injured posterior ethmoidal air cells and the sphenoidal sinus, the clinical presentation also includes the orbital apex syndrome.
Septic encephalopathy is common for this condition; cerebral meninges can also be involved in the pathological process. Diagnosis verification and differential diagnosis with the orbital abscess and cavernous sinus thrombosis require performing CT scanning or preferably MRI.
Cavernous sinus thrombosis is caused by septic embolism of cerebral sinuses and is often bilateral.
Fever, chills, marked changes in mental status, and elevated leukocytes are all signs and symptoms of brain involvement. The impeded venous outflow from the orbit is associated with marked chemosis, dilated episcleral veins, increased ocular pressure, optic disk congestion, tortuosity and congestion of retinal veins, and exophthalmos. Dysfunction of trigeminal nerve branches I and II and sequential palsy of the abducent, oculomotor, and trochlear nerves are also classic signs of cavernous sinus thrombosis.
Cavernous sinus thrombosis is characterized by rapid progression leading to loss of consciousness and coma.
3.4.3.2 Treatment
Purulent complications involving orbital soft tissues require urgent intensive therapy; it is only preseptal cellulitis that requires using conservative treatment. In all other cases, urgent opening and drainage of paranasal sinuses by an otolaryngologist and a maxillofacial surgeon is required in addition to intravenous injection of broad-spectrum antibiotics (co-amoxiclav, ceftriaxone, meropenem), anticoagulation, and stabilization of blood pressure. Taking this into account, it seems most reasonable that these patients are admitted to the otolaryngology or the maxillofacial surgery unit.
The following measures may be needed in addition to surgical drainage of the primary focus such as sinusitis:
Opening of subperiosteal abscess via the exo- or endonasal approach
Canthotomy, cantholysis, and orbitotomy aimed at orbital decompression or opening and drainage of the orbital abscess
It is clear that treatment of orbital fractures, especially using grafting material, should be postponed in these cases [377].
The vision and oculomotor functions may return entirely to normal provided that treatment of the infectious state was initiated in a timely and thorough manner [378].
3.4.4 Late Implant Infection
The reasons for late implant infection include dental surgeries, rhinoplasty, implant migration into the paranasal sinus causing a sino-orbital fistula, dacryocystitis caused by medial displacement of the graft, drug abuse, or acute respiratory viral infection [379]. According to the published data, infection of a porous implant inevitably causes explantation [202, 270, 334]. Staphylococcus aureus and epidermidis are the bacterial species typically detected by microbiological examination in this case.
3.4.5 Optic Neuropathy
Trauma-induced optic neuropathy can result in either partial or complete vision loss without external or primary ophthalmoscopic signs of damage to the globe after the trauma [24].
Blindness after optic nerve trauma can be caused by the direct impact of the kinetic energy of a wounding agent, by rupture of pial sheath vessels, or by development of the compartment syndrome secondary to retrobulbar or subperiosteal hemorrhage. Since the optic nerve is rarely affected in patients with the classical blow-out fracture of the orbital floor, diagnosis and treatment of neuropathy caused by blunt orbital trauma is provided in the subsequent chapters of this handbook.
Loss of central vision after osteoplasty indicates that the optic nerve or vessels feeding it were compressed by the implant, or ischemic optic neuropathy resulted from uncontrolled intraoperative arterial hypotension [237, 380]. Retrobulbar or subperiosteal hemorrhage and marked edema of orbital fat can also occur because of the severity of surgical wound [381, 382]. Fortunately, the risk of this disastrous complication is less than 0.07 % [84].
Urgent orbital decompression, removal of the implant compressing the nerve, and hematoma drainage combined with megadose glucocorticoid therapy may lead to some improvement of visual functions [202, 381].
Prevention of postoperative vision impairment includes elimination of excessive pressure exerted onto the eye and the optic nerve during osteoplasty, periodic intraoperative measurement of blood pressure to monitor for severe arterial hypotension, the use of the smallest implant that is sufficient to close the defect for subperiosteal implantation, and reliable fixation of the implant. If a compression bandage is used, it should be removed the next morning to test visual acuity and pupillary responses and to perform ophthalmoscopy.
Special care is needed when performing tamponade of the maxillary sinus, since this procedure may significantly increase the intraorbital pressure [237]. The development of compressive optic neuropathy can be caused by deliberate or accidental placement of a hemostatic sponge in the posterior portions of the orbit after surgical repair [383].
Meanwhile, it seems unlikely that the optic nerve can be directly damaged with surgical instruments, since the orbital floor is characterized by 15° elevation and S-shaped profile that prevent accidental placing raspatory into the deep orbital compartments. Furthermore, the distance between the infraorbital margin and the orbital apex of 45 mm also plays a protective role [12]. There is no reliable evidence demonstrating that manipulations in the orbit cause increased intraorbital pressure that is dangerous for blood circulation [380].
As opposed to the commonly held belief, bone fragment repositioning does not increase ocular pressure. This was demonstrated by the use of intraoperative tonometry reported by Paton et al. [384]. Placement of large implants during late orbital reconstruction may cause short-term ophthalmic hypertension, but it does not affect the ocular functions at all [93, 380, 385].
3.4.6 Diplopia
3.4.6.1 Definition and Classification
It is reasonable to start describing one of the most severe complications of both the fracture itself and its surgical management by discussing which type of diplopia should actually be regarded as a complication. The diplopia should also be graded as mild, moderate, or significant.
Theoretically, osteoplasty of the orbital floor should not cause diplopia. However, since not every diplopia type is an indication for surgical management, not every postoperative diplopia should be regarded as a complication.
In particular, diplopia in extreme positions of gaze (referred to as mild by Hammer and Prein [386]) is not a complication and does not require treatment [12].
Primary gaze diplopia is considered severe and requires therapy.
It would seem reasonable to consider upward-gaze diplopia to be of moderate severity, since the absence of diplopia in the lower visual fields allows the patient to walk and perform visual activity at a short working distance. However, there are a number of occupations for which upward-gaze diplopia interferes with professional competency, hence in this case would be considered as being severe.
Synonymous terms, such as “diplopia in functionally important gaze directions,” “disturbing diplopia,” and “clinically significant diplopia,” seem to be more appropriate for these cases25.
When performing mathematical and statistic calculations, researchers can use the gradation of diplopia and oculomotor disorders proposed by Grant et al. [387].
Degree of oculomotor disorders:
0—The range of movements is identical to that of a healthy eye.
1—For the maximum supraduction, the inferior limbus of the damaged eye is diverged from that of the healthy eye by less than 1 mm.
2—1–2-mm divergence.
3—The divergence fluctuates within 2–3 mm.
4—Divergence of the injured eye is over 3 mm.
3.4.6.2 Degree of Diplopia
0—No diplopia.
I—The horizontal divergence angle at which diplopia emerges is 45° or more.
II—The horizontal divergence angle at which diplopia emerges is 15–45°.
III—The angle of gaze divergence is less than 15°.
IV—Diplopia in primary gaze position.
3.4.6.3 Epidemiology of Diplopia
Both a surgeon and a patient should bear in mind that even a perfect surgery can be accompanied by emergence or aggravation of the already existing diplopia early after the intervention. It is transient diplopia that requires no special treatment [8, 213].
One should not make any judgments as to the degree of severity of diplopia for at least 30 days after surgery. Complete regression of diplopia and concomitant paresthesia of the infraorbital nerve may take 2–6 months [175, 320, 386].
3.4.6.4 Risk Factors of Persistent Diplopia
The obvious risk factors of persistent postoperative diplopia include extensive (e.g., inferomedial) fracture [76], its spread to the so-called deep orbit defined as being behind the inferior orbital fissure which is an anatomical border of the orbital floor [390], tamponade of the maxillary sinus26 [350], advanced age of a patient [75], and delayed surgical management [118, 238, 391]. Full ocular motility is obtained in 80 % of patients operated on during the first week after trauma, 50 % if operated on during the second week, and in less than 25 % of patients if the surgery is delayed longer than 2 weeks.
The mechanisms of transient or permanent diplopia in patients with blow-out fracture can vary [390].
Muscle edema and/or hematoma are clearly visualized on high-resolution CT scans [99]. The negative traction test result facilitates diagnosis. The outcome is complete recovery without surgical intervention.
Muscle entrapment in the fracture site [118] is observed in only 5–10 % of cases [387] but is an unfavorable prognostic factor associated with the risk of persistent diplopia. The contact between muscle belly and the bone at two points seen in sequential CT scans is the CT sign of muscle entrapment [345].
Fibrosis or entrapment of fat and connective tissue interconnections of the orbit [107, 118, 392], which are a passive component of the oculomotor system and comprise the integral locomotor system together with the muscles [106].
Dislocation of extraocular muscles accompanying enophthalmos and hypoglobus changes their traction vector and results in muscular imbalance and diplopia. The hypothesis relies on cases when diplopia disappeared after surgical correction of hypoglobus without any interventions on extraocular muscles [387].
Volkmann’s ischemic contracture in patients with trapdoor fractures [393]. Smith et al. [394] performed direct intraoperative measurements to demonstrate a significant increase in pressure in the sheath of the inferior rectus entrapped at the fracture site. The microcirculation in muscular tissue is impaired, resulting in the development of the “Volkmann’s ischemic contracture.”
This mechanism most typically occurs in hypotensive patients with pronounced edema of orbital tissues. As opposed to the trapdoor fracture, swollen tissues in patients with an extensive bone defect can migrate to the sinus, thus preventing a significant increase in orbital pressure.
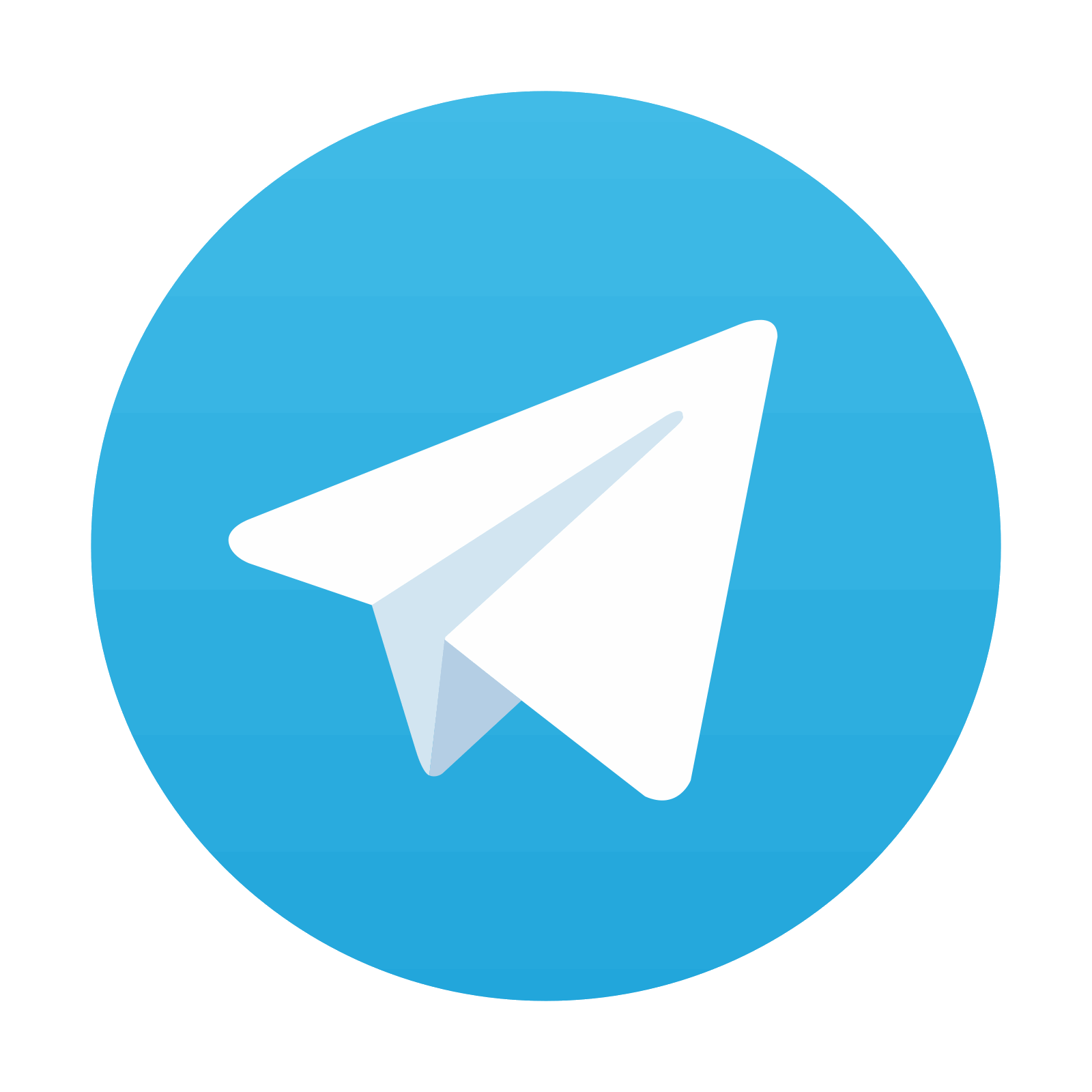
Stay updated, free articles. Join our Telegram channel
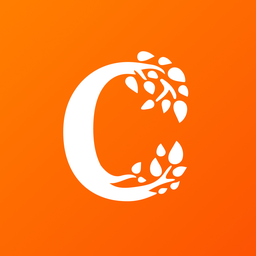
Full access? Get Clinical Tree
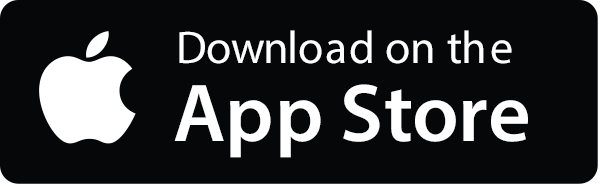
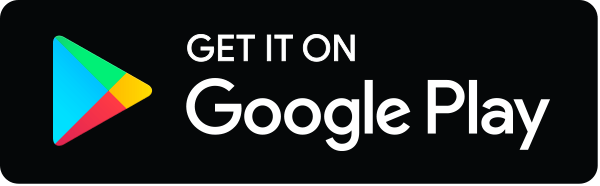