Ophthalmic Photodynamic Therapy
Edgar L. Thomas
Wendy J. Snyder
Stephen Rychnovsky
John S. Hill
Photodynamic therapy (PDT) for the treatment of choroidal neovascularization (CNV) associated with exudative age-related macular degeneration (AMD) and ocular tumors have evolved from the earliest concepts of light activated dyes for treatment of cutaneous cancers in the early 1900s. PDT is a binary therapy comprising two individually nontoxic components that can mediate a focal effect when they are combined. These two components are (i) the systemic or local administration of a photosensitizing drug and (ii) the subsequent activation by light of that drug in the target tissue.1 Photodynamic activation of the photosensitizer results in the transfer of energy to molecular oxygen, resulting in a flux of reactive oxygen species, and possibly other radicals, that damage multiple targets, such as proteins and lipids, and disrupt cellular processes leading to cell death.
The concept of PDT for the treatment of patients with malignant disease dates from the early 1900s with the work of Jesionek and von Tappeiner,2 who utilized topical application of eosin in combination with sunlight to treat skin cancer. The first photodynamic studies using hematoporphyrin were carried out by Hausmann3 several years later. In the 1940s, Auler and Banzer4 and Figge et al.5 demonstrated the affinity of various porphyrins for hyperproliferating tissues. In attempting to improve the localization and photodynamic properties of hematoporphyrin, Lipson and Baldes6 developed a complex mixture of porphyrin species called hematoporphyrin derivative. The first detailed clinical report of PDT with hematoporphyrin derivative was published in 1976 by Kelly and Snell,7 who treated a patient with recurrent superficial bladder carcinoma. The clinical development of PDT then was systematically pursued by Dougherty and others.8
By the late 1990s, a significant number of patients worldwide had received PDT for malignancies in a variety of sites, including the skin, the lung, the esophagus, the bladder, the brain, and the eye, and regulatory approval had been granted for the use of PDT in treating varying types of malignancies. Mechanistic studies suggested that tumorous tissues could be eradicated or undergo significant regression without harming adjacent healthy tissues. The tumor responses seen in the studies were found to be caused, in part, by a direct phototoxic effect on the tumor cells, and also by accompanying damage to proliferating endothelial cells in the tumor associated vasculature. On noting this vascular damage, there was a subsequent expansion of the modality to treatment of nontumor neovascular proliferations in the eye, such as CNV associated with AMD. Nonclinical and clinical studies had previously demonstrated the ability to cause vascular occlusion of proliferating neovessels under the fovea while preserving and/or improving visual acuity of patients afflicted with the disease.
The following sections consider some of the key determinants of efficacy of PDT treatment with specific reference to the treatment of neovascular lesions in the eye and also discuss the two photosensitizers that have been most widely used in this field; Visudyne, which has received worldwide regulatory and marketing approval, and Photrex, which has been tested clinically as a treatment for AMD but has not yet been approved.
Photophysics
When a photon of energy is absorbed by a photosensitizing molecule in its ground state (0S), a variety of reactions may take place (Table 1). One of the reactions involves the photodynamic process. Thus, the action of the photosensitizer in PDT is to absorb photons of the appropriate wavelength and intensity sufficient to elevate the photosensitizer to an excited state. For a molecule to absorb electromagnetic energy and advance from a stable ground state of energy to an electronically excited state, two criteria must be satisfied. First, the energy of the photon must match the energy difference of the allowable excited states of the electron bond in the molecule. For visible light, this corresponds to the electrons in the outer valence levels. Second, there must be a specific interaction of the electric vector of the light quantum, which induces the transition of the electron from its ground state orbital to its new orbital in the excited state. The absorption of the light quantum then raises the energy by promoting the electron to a higher energy state (singlet, 1S).
TABLE 1. Photodynamic Process | ||||||||||||||||
---|---|---|---|---|---|---|---|---|---|---|---|---|---|---|---|---|
|
The excited molecule then may de-excite by a variety of competitive pathways, as shown in Table 1. The pathway chosen is independent of the photon used for excitation. The ability of a given wavelength of light to drive a photochemical reaction is determined by the absorption characteristics of the molecule.
In leading to the photodynamic process, one of the ways in which an excited molecule in the singlet state may de-excite is through a nonradiative process called intersystem crossing, in which the electron spin reverses, leading to an excited triplet state (3S). The triplet state, also called a metastable state, has a lower energy than the corresponding singlet state because of the repulsive nature of the spin–spin interaction between the electrons of the same spin. The photosensitizer, in its excited triplet state, then can undergo two kinds of reactions. It can react directly with substrates to form radicals or radical ions (type I reaction). Alternatively, it can react with endogenous ground state oxygen (3O2) by means of an energy transfer mechanism in producing excited oxygen species, such as singlet oxygen (1O2). This latter reaction is known as the photodynamic reaction and is a type II reaction. The excited oxygen species are highly reactive toward many biomolecules and, hence, toxic to cells and tissues.
Photobiology
The photobiologic effects in the tissue are driven by the absorption of light by the photosensitizer. The probability of photon absorption (extinction coefficient, ep;cm-1 M-1) depends on the treatment wavelength. The absorption coefficient of the photosensitizer in tissue, uap, thus is given by uap = ep[C], where [C] is the molar concentration. For treatment with a monochromatic source at a specific wavelength, the rate of energy absorption by the photosensitizer is dependent on the product of the effective light fluency at a specified irradiance, the photosensitizer concentration, and the photodynamic efficiency in the targeted tissue. The newer, second-generation photosensitizers have singlet oxygen quantum yields up to 90%, leading to high efficiencies compared with first-generation compounds.9
For most photosensitizes, photodynamic effects are oxygen dependent (type II reactions). Cellular and tissue damage mediated in this fashion occurs close to the production of the excited oxygen species. Singlet oxygen (1O2) diffusion distance in a cellular environment has been estimated by Moan10 to be approximately 0.1 μm. Therefore, cell damage mediated by 1O2 occurs close to its site of generation and can affect most cellular components. Photosensitizers have been shown to be associated with or localized to membranous organelles, such as plasma, mitochondrial, lysosomal, and endoplasmic reticulum membranes.11
The most easily and rapidly discernible acute effects of PDT in vivo are of a vascular nature.12,13 Variable responses to PDT have been reported in poorly vascularized pathologies. The development of microscopic and macroscopic tissue damage has been well described for a number of photosensitizers, including Photofrin, benzoporphyrins, purpurins, and phthalocyanines.12 The interval between initiation of damage and vascular occlusion may vary from tissue to tissue and with different photosensitizers, but eventual vessel occlusion appears to be the general phenomenon accompanying PDT12
Major determinants for vascular therapeutic damage appear to be the level of circulating photosensitizer and binding to endothelial cells and erythrocyte membranes.12 Vascular occlusion can be induced on light exposure of tissues shortly after drug injection. With greater time allowed between photosensitizer administration and illumination, however, light doses necessary for vascular occlusion vary greatly.
Thus, the acute, lethal effects on cells and tissues depend on the localization of the photosensitizer on or within the cells, the photodynamic efficiency of the compound in that environment, the absorbed light dose reaching the targeted area, and the kinetics of vascular occlusion and oxygen supply.
Devices and Dosimetry
Photodynamic therapy typically requires a light source, but not necessarily a laser system, coupled to light delivery systems pertinent to the application. Devices include broadband light from incandescent or arc lamps or monochromatic light from lasers in the visible and near infrared region. The activating light can be delivered either directly from the source device or via fiber optics coupled to the device. The fiber optics can be composed of various specialty ends, which distribute light in a pattern advantageous to the tissue geometry. One distinct advantage of fiber optics is to allow clinicians to direct light in a minimally invasive manner during an intraoperative procedure. For applications of PDT in posterior and anterior segments of the eye, the activating light can be delivered via either fiber optics or optical devices coupled and adapted to diagnostic slit lamps (Fig. 1) to allow for transpupillary light delivery.
![]() FIGURE 1. A 664-nm semiconductor diode laser adapted for Haag Streit Slit Lamp Delivery (Miravant Systems, Inc., and Iris Medical Instruments, Inc.). |
The optical accessibilities and properties of the eye are compatible with PDT. Visible light is transmitted to the retina with little absorption loss.14 All anterior segment components essentially are nonscattering, thus allowing their functioning as high-quality optical elements. In contrast, the sclera is highly scattering for the light reflecting from the retina and choroid. The effect of its back-scattering into the eye is diminished greatly by the pigmented epithelial layer.
PDT typically is performed with lower irradiances than those used in thermal laser photocoagulation, such as 150 to 200 mW/cm2, levels at which photochemistry predominates over thermal mechanisms. Mild hyperthermia may be produced at greater irradiances (e.g., >600 mW/cm2). If the optical power densities and energy densities are high enough, then a significant temperature increase can be produced in the exposed tissue, as in laser retinal photocoagulation. Synergism between PDT and hyperthermia has been reported by numerous investigations.15,16
Practical considerations and differences in performing PDT treatment via the slit lamp versus thermal laser photocoagulation are the use of lower irradiance and power levels, longer durations of light application, and increased spot sizes. Because the duration of light ranges from seconds to minutes, proper alignment of the activating light beam must be maintained. Because the intensity of the light therapy is low, patients appear to tolerate the prolonged light treatment. In patients unable to fixate because of poor vision in the fellow eye, retrobulbar anesthesia for akinesia occasionally may be necessary. Typical powers required for PDT range from 5 to 50 mW, covering beam diameters of 0.5 to 3 mm spot sizes. Table 2 provides definitions and abbreviations for light terminology used in the treatment.
TABLE 2. Light Terminology: Definitions and Abbreviations | |
---|---|
|
Photosensitizers
Administration of the photosensitizer, followed at the appropriate time by light application at a specific wavelength, can result in effective therapeutic treatment of the targeted tissue. The factors governing the effectiveness of a given photosensitizer are numerous and depend in part on its physiochemical and photophysical properties, as well as the consequent molecular events following light activation (see below). As previously noted, the absorption spectrum of the drug dictates the wavelength of the illumination beam that may be used for PDT. In general, the wavelength selected coincides with an absorption maximum of the photosensitizer. Other important considerations include the metabolism, pH, pharmacokinetics, and route of administration of the photosensitizer. After photosensitizers are administered to a patient, they exhibit a relative affinity for vascular and hyperproliferating tissues. This relatively selective accumulation, as well as the capacity for focal light activation, provides the basis for PDT over nonselective therapies, such as thermal laser photocoagulation.
Several major classes of photosensitizers have been used in vitro and in animal models for mechanistic studies of photodynamic injury and to develop an optimal agent for human disorders. Among these agents, porphyrins, benzoporphyrins, purpurins, phthalocyanines, bezophenoxazines, and xanthenes have been used for ophthalmic applications.17
Photofrin (porfimer sodium) is the photosensitizer that has been studied most extensively in oncologic applications of PDT.18 Photofrin and its predecessor, a hematoporphyrin derivative, are prepared from hematoporphyrin and essentially are complex mixtures of oligomeric esters and ethers of hematoporphyrin. Despite the relatively good success of Photofrin PDT in treating various cancers of the bladder, lung, and esophagus, disadvantages with this first-generation photosensitizer have spawned an interest in producing more photodynamically efficient photosensitizers with less cutaneous phototoxicity.18
In recent years, a number of second-generation photosensitizers have been developed for use in PDT. Both hydrophobic and hydrophilic photosensitizers are being developed and proposed for treatment. Developments made in drug carrier systems, such as liposomes, lyophilization, and lipid emulsions, have made concerns of hydrophobic drug delivery less important while possibly offering some advantages over hydrophilic compounds.19 Two second-generation photosensitizers that have undergone extensive clinical testing in the ophthalmic field are Visudyne (benzoporphyrin derivative mono-acid ring A; BPD) and Photrex (tin ethyl etiopurpurin; SnET2).
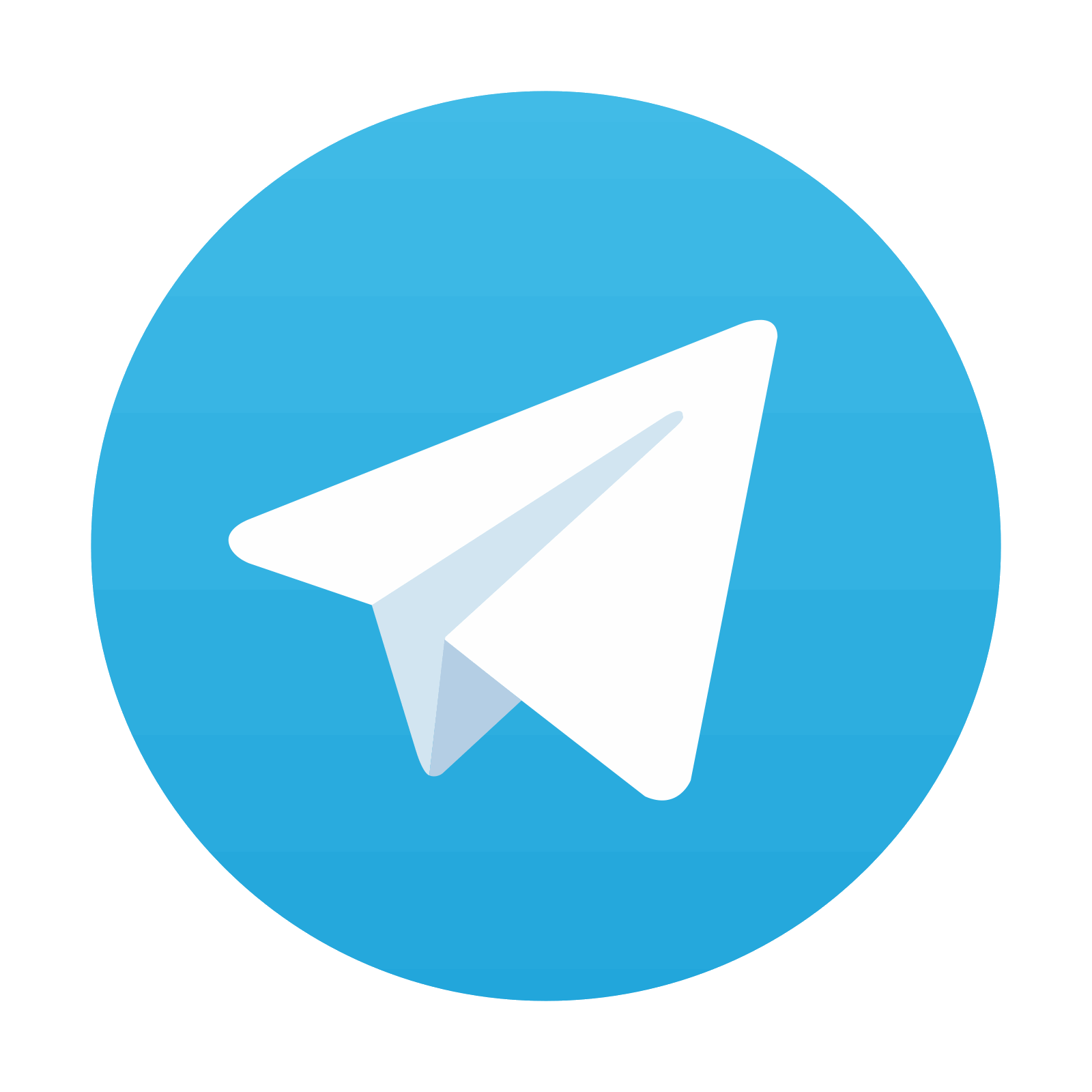
Stay updated, free articles. Join our Telegram channel
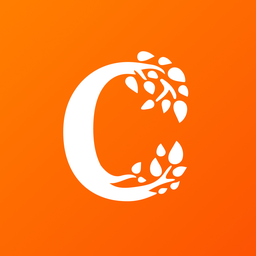
Full access? Get Clinical Tree
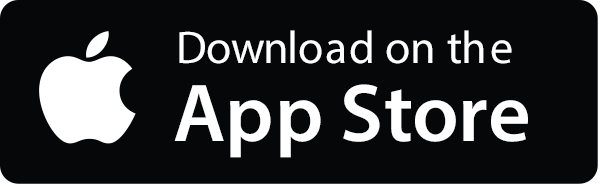
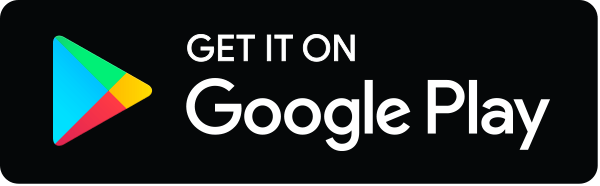