Key points
- •
Cochlear device implantation (CDI) remains the only reliable option for auditory communication rehabilitation in cases of severe and profound sensorineural hearing loss (SNHL) where the site of lesion is outside the central auditory processing stream.
- •
Cochlear implants (CIs) sample the acoustic environment, process the input signal into discrete frequency bands, compress the amplitude into an electrically useable range, and then stimulate the residual neural elements in a tonotopic manner to reproduce the frequency- and amplitude-analyzing capability of the cochlea.
- •
CIs represent the most successful neural prosthesis in clinical use and have a long and interesting history that has led to the modern devices that are currently available. Further refinements of the existing current iteration of these devices and the development of novel technology hold promise to continue to improve and benefit patient experience.
Introduction
Most patients with hearing loss significant enough to result in social dysfunction can be treated with nonsurgical interventions. In many instances, environmental manipulations are sufficient to improve auditory communication, typically by way of improving the signal-to-noise ratio or relative amplification. Examples include minimizing ambient noise such as avoiding crowded or noisy listening situations, selective seating such as sitting closer or with the better ear near important sound sources, or the use of frequency-modulated or infrared (IR) devices. When these manipulations are insufficient, amplification of the acoustic environment can be used. This amplification may take many forms including personal listening devices or conventional hearing aids. In those with conductive hearing loss that is not amenable to conventional amplification that uses air conduction mechanisms, bone conductive solutions are available including osseointegrated and active middle ear implants.
CDI remains the only reliable option for auditory communication rehabilitation in cases of severe and profound SNHL where the site of lesion is outside the central auditory processing stream. CIs sample the acoustic environment, process the input signal into discrete frequency bands, compress the amplitude into an electrically useable range, and then stimulate the residual neural elements in a tonotopic manner to reproduce the frequency- and amplitude-analyzing capability of the cochlea. CIs represent the most successful neural prosthesis in clinical use and have a long and interesting history that has led to the modern devices that are currently available. Further refinements of the existing devices and the development of novel technology hold promise to continue to improve and benefit patient experience.
Introduction
Most patients with hearing loss significant enough to result in social dysfunction can be treated with nonsurgical interventions. In many instances, environmental manipulations are sufficient to improve auditory communication, typically by way of improving the signal-to-noise ratio or relative amplification. Examples include minimizing ambient noise such as avoiding crowded or noisy listening situations, selective seating such as sitting closer or with the better ear near important sound sources, or the use of frequency-modulated or infrared (IR) devices. When these manipulations are insufficient, amplification of the acoustic environment can be used. This amplification may take many forms including personal listening devices or conventional hearing aids. In those with conductive hearing loss that is not amenable to conventional amplification that uses air conduction mechanisms, bone conductive solutions are available including osseointegrated and active middle ear implants.
CDI remains the only reliable option for auditory communication rehabilitation in cases of severe and profound SNHL where the site of lesion is outside the central auditory processing stream. CIs sample the acoustic environment, process the input signal into discrete frequency bands, compress the amplitude into an electrically useable range, and then stimulate the residual neural elements in a tonotopic manner to reproduce the frequency- and amplitude-analyzing capability of the cochlea. CIs represent the most successful neural prosthesis in clinical use and have a long and interesting history that has led to the modern devices that are currently available. Further refinements of the existing devices and the development of novel technology hold promise to continue to improve and benefit patient experience.
History of cochlear implant development
The history of CDI spans over 60 years and has seen multiple iterations of the devices and speech processing strategies utilized, although the initial use of electrical audition preceded CDI by almost 200 years. Alessandro Volta performed the first documented electrical stimulation of the auditory system in 1790 when he applied a large voltage across his own ears and was able to generate auditory percepts he described as crackling or bubbling. Later experiments applied alternating currents (Duchenne of Boulogne) as well as various charges, polarities, and intensities (Brenner). Weaver and colleagues (1930) described electrical signals from the feline cochlea that closely resembled the input stimulus waveform with the implication that it might be possible to replicate this result with electrical signals.
Djourno and colleagues (1957) implanted an electrode coupled with a receiver coil into a patient having undergone resection of the distal cochlear nerve during the treatment of an extensive cholesteatoma and were able to stimulate the apparatus with an external coil for several months. Amazingly, this patient was able to develop sound awareness and simple word recognition. William House began his pioneering work in the early 1960s, inspired by the work of Djourno and Eyries, starting with the implantation of either simple wires, wires with ball electrodes, and even simple arrays into the scala tympani. This early work in partnership with Jack Urban eventually resulted in the development of a commercially available implantable device in 1972 with clinical trials beginning the following year.
Although met with considerable skepticism and resistance from the basic science community including leading neurophysiologists and otologists, the validity of direct electrical stimulation of auditory nerve fibers as a rehabilitative strategy was confirmed in 1977 by a team commissioned by the National Institutes of Health that evaluated the outcomes from patients implanted with single-channel devices. In a major advancement, Clark developed a multichannel CI, which was able to produce open-set word recognition. Following the Food and Drug Administration (FDA) approval of the single-channel CI, multichannel devices soon replaced the single-channel device because of better frequency spectrum percepts and open-set word recognition. Based on these developments, multiple multichannel CI devices are available with varying numbers of electrode contacts, electrode lengths, electrode widths, and electrode positioning technologies from 3 device manufactures (Advanced Bionics, Cochlear, and MED-EL).
Environmental speech formant processing and electrode activation strategies developed in parallel to that of CI design over the past several decades. The initial single-channel CIs used simple sinusoidal currents to drive neural responses, whereas multichannel CIs used simultaneous stimulation of discrete locations of the modiolus in a tonotopic manner, this being termed compressed analog strategy. Although this latter stimulation paradigm allowed for limited open-set word recognition, the spread of the temporally synchronous current resulted in issues of channel interaction. Other early speech processing strategies included feature extraction (called the PEAK strategy) and the use of multiple filter banks (Spectral Peak Extraction or SPEAK). In 1991, Wilson and colleagues introduced the continuous interleaved sampling (CIS) strategy, which demonstrated significantly improved open-set word recognition when compared with previous analog strategies. Today, all commercially available pulsatile strategies are based on CIS.
Contemporary cochlear implants and targets for innovation
Successful auditory system stimulation resulting in meaningful perceptions requires several technological and biological components, all of which are targets for continued innovations. Acoustic stimuli must be detected and captured (microphone), processed (speech processing software and circuitry), turned into electrical signals (coil, receiver/stimulator) that are delivered to the spiral ganglion neurons (SGNs) (electrode array), transduced into action potentials, and delivered to the central auditory processing stream. Carlson and colleagues (2012) provide a review of the components of the modern CI. Briefly, most CIs consist of an external device worn as a behind-the-ear device incorporating one or more microphones that convert acoustic energy into an analog signal. This signal is then typically digitized, compressed, filtered, and encoded into a signal that is used to drive SGN stimulation. This code is transmitted through the skin using radiofrequency signals to a completely subcutaneous signal receiver that drives intracochlear electrode activation. A variable number of electrodes are encased within a carrier (commonly referred to as the electrode or electrode array), the length of which varies according to the specific device. SGNs are directly driven with electrical voltage delivered by the electrode array to generate action potentials, which are conducted to more central locations in the auditory system. Most CIs also have a return/inactive (ground) electrode that is either part of the body of the receiver/stimulator or a separate lead implanted in the soft tissues around the ear, typically deep to the temporalis muscle. The active electrodes (intracochlear) can be activated in 2 main configurations: monopolar and bipolar. In monopolar stimulation, each intracochlear active electrode uses the extracochlear inactive electrode as the current return. In the bipolar mode, 2 neighboring electrodes form an active/inactive pair. Each mode has its advantages and disadvantages, which are beyond the scope of this review.
The premise of CDI is simple: patterned electrical stimulation of cochlear afferent fibers. Thus both the processing of the acoustic signal (electrical stimulation code) and the neural responses are critical. Unfavorable electrical stimulation or neural response characteristics results in poor perceptual outcomes. The residual neural elements and their health as well as the ability of the CI to deliver high-fidelity electrical stimulation are the basic substrates of contemporary cochlear implantation. Neuronal health has received considerable attention in recent years with several works demonstrating that reduced intracochlear damage with device placement, presumably resulting in improved neuronal survival, is associated with improved speech perceptual abilities.
Remote CI programming, totally implanted devices, improved neural health and survival through targeted drug therapy and delivery, intraneural electrode placement, electroacoustical stimulation and hybrid CIs, and methods to enhance the neural-prosthesis interface are evolving areas of innovation reviewed in this article.
Totally implantable cochlear implants
Totally implanted cochlear implants (TICIs) may have advantages when compared with current commercially available devices, which require an external device that couples to the implanted receiver/stimulator. External devices are exposed to the environment, which may render them more vulnerable to damage from extremes of temperature, moisture, and dislodgement. In addition, implantees typically remove the external device when water exposure is likely (eg, bathing, swimming) or when perspiration is great (vigorous exercise) and thus are off-line during these activities. Although the size and profile of current external devices are smaller and less conspicuous than those of earlier-generation devices, they are visible (more so than modern behind-the-ear hearing aids), which may not be desirable to many potential candidates for social reasons. There are several technical barriers to implantation of a TICI including power source management, environmental sound detection, and management of component breakdown. Contemporary CIs are powered via electromagnetic induction using radiofrequency signals via the coil of the external device and antenna of the receiver/stimulator. Any TICI would need to be powered internally, likely with the use of a rechargeable battery. Batteries need to be able to recharge quickly, hold enough charge to power the CI for about a day, not generate significant heat, and have a very low chance of leaking potentially dangerous battery chemicals even in the event of battery failure. At this time, all rechargeable batteries eventually fail to hold significant charge and need to be replaced (a strategy used with pacemakers). In addition, current CIs use an external device worn behind the ear that houses one or more microphones and provides a largely unfettered access to the acoustic environment and takes advantage of the filtering properties of the head. A TICI needs to overcome the more limited direct access to sound sources. Options include microphone placement subcutaneously in the external auditory canal or behind the ear or using the tympanic membrane and/or ossicular chain as a microphone directly. The speech processor and related electrical components also needs to be implanted. It is likely that with the increased number of components implanted, an explantation strategy will need to be devised because component failure becomes more likely. It is also probable that TICI will need some type of external hardware for battery recharging, programming, and switching between programs. It may also be desirable to allow the TICI to be powered and stimulated using a conventional external device. Briggs and colleagues (2008) published the first report using a TICI system in 3 subjects. The investigators termed the use of the TICI alone as invisible hearing. The devices used a subcutaneous microphone near the radiofrequency coil, lithium ion battery, and the ESPrit 3G (Cochlear Corporation, New South Wales, Australia) external sound processor for use in a conventional mode. Results indicated that the devices can be safely implanted and all 3 subjects reported benefit when using the devices in the invisible hearing mode. However, the subjects scored higher in measures of consonant-nucleus-consonant word testing in quiet and City University of New York sentence scores in noise when using the devices in conventional mode with the external speech processor. With continued improvement, it is likely that TICI will become a viable and common device option for patients.
Telemedicine and remote programming of cochlear implants
After CDI, the brain learns to use the encoded electrical stimulation to extract information about the acoustic environment; this is a dynamic process with continued improvements being seen years after the initial device activation. Each electrode in the array must be tuned to the response properties of the region that it stimulates, which typically involves determining the psychophysical threshold and the maximum comfortable level of stimulation (also known as T and C levels, respectively). Over time, changes in T and/or C levels, individual electrode failures or extrusions, and nonauditory stimulations (eg, facial nerve stimulation) require reprogramming the speech processor. CI programming has traditionally been performed in the clinical setting by an implant audiologist using proprietary equipment and software. This programming has required health care encounters at dedicated CI program centers, often necessitating a travel requirement for patients and their families. Modern telecommunication technologies may offer an approach to programming where patients and their CI team can work together to maximize each user’s performance without a physical visit to the implant center. This approach can offer specific benefit to patients with limited access to transportation or who live in remote areas. Several recent reports document the safety and efficacy of remote programming. Ramos and colleagues (2009) describe a fairly simple setup for remote programming based on software for video conferencing, computer operating systems, and standard CI programming software and hardware. In their experimental setup, a remote unit equipped with all required programming equipment and attended by a local representative interacted with a remote location equipped with similar computing equipment and programs. The remote computer was able to control the local computer and thus run the programming software. In their study of 5 subjects who were programmed both with standard and remote programming sessions, they found that remote programming was safe and was not statistically different from standard programming. McElveen and colleagues (2010) also demonstrated the safety and noninferiority of remote programming in 7 patients compared with 7 matched controls using a setup similar to that of Ramos and colleagues (2009). Wesarg and colleagues (2010) evaluated 70 subjects over several different implant centers using a variety of technology and found no significant difference in map characteristics between remote and local programming sessions. One common finding in these 3 studies is the presence of monitoring personnel to ensure the safety of the subject being programmed by watching for signs of painful stimulation and ensuring that there are no communication issues between the remote programming audiologist and the subject. Patient and health care professional satisfaction with remote CI programming in these and other studies has been quite high, with one recent study reporting that greater than 96% of respondents were satisfied with remote programming sessions and 100% reporting that they would use remote programming in the future. These studies demonstrate that remote programming is safe and feasible and may offer an opportunity for better access and possibly improved outcomes for patients undergoing CDI who live at a considerable distance from their implant center.
Optical neural stimulation and optical cochlear implants
Contemporary hearing rehabilitation currently relies on 2 main modalities: acoustic stimulation to the cochlea and electrical stimulation of remaining cochlear nerve afferents. Both strategies have advantages and limitations. Acoustic stimulation relies on the presence of mechanoacoustic stimulation of the cochlea, typically with amplified and filtered signals (eg, conventional hearing aids) and necessarily relies on cochlear functions including the biomechanics of the basilar membrane and organ of Corti as well as the physiology of the inner and outer hair cells. In the case of conventional hearing aids, this requires a patent external auditory canal that can tolerate the placement of the hearing aid. In severe cases of SNHL, these biomechanical and physiologic properties are deranged to the point where mechanoacoustical stimulation does not provide the subject with a hearing benefit. In many of these patients, a reduced but viable population of SGNs remain that can be driven with nearby electrical voltage changes and this provides the neural basis for electrical hearing via CDI. With modern CI electrode arrays and stimulation paradigms, CI users are commonly performing at perfect or near-perfect levels on word recognition tests including hearing in noise situations. However, despite these results, many patients continue to report difficulty hearing in noisy environments and with music perception. As reviewed by Richter and colleagues (2013), although there are at most 22 electrodes, only 4 to 7 channels are truly independent versus the estimated 30 to 50 channels in subjects with normal hearing. The main problem is thought to be the spread of current (spread of excitation) away from active electrodes. This spread of excitation may degrade the specificity of the neural elements being stimulated through channel interaction or cross talk. Channel interaction is an example of how the spread of the excitatory currents degrade the neural percepts with CIs. It is likely that increasing the number of independent channels will improve listening in noise or music appreciation. Virtual channels, created by steering current between 2 electrodes, and bipolar electrode stimulation have been used to decrease channel interaction but have yet to result in significant improvement over more traditional stimulation methods.
The use of photons as the energy source for neural stimulation has been proposed as one mechanism to more specifically stimulate neural elements (reviewed in Richter and colleagues, 2013; Eshraghi and colleagues, 2012; Jeschke and Moser 2015 ; and Moser, 2015). Fig. 1 provides a demonstration of how optical stimulation may provide more precise stimulation when compared to current cochlear implant devices. The precision that light stimulation may offer could allow for the creation of more focal stimulation and thus more independent channels of information flow. Light energy has been found to excite many different types of tissues including peripheral nerve, cortical cells, cardiomyocytes, and isolated neurons. In addition, the light energy could be delivered via local light sources such as miniature light-emitting diodes or could be transported via special wave guides. There are several proposed mechanisms for how light can stimulate neural tissues: (1) photoactivation of light-gated ion channels (optogenetics), (2) thermal stimulation of heat-gated ion channels (thermogenetics), (3) direct activation through alterations in local plasma membrane electrical properties, (4) uncaging of neurostimulatory compounds, and (5) modulation of intracellular calcium metabolism. Optical stimulation mechanisms, specifically optogenetic and IR light stimulation, have received the most attention.
Optogenetics and thermogenetics require the expression of ion channels in the tissue to be excited, which in the case of hearing loss and CIs in particular, are the SGNs. Since the discovery of channelrhodopsin (ChR) 1 and 2, the expression of these channels has become a popular method for neural stimulation throughout the neurosciences. The molecules function as transmembrane light-gated ionotropic channels. Several ChRs have been developed with a variety of kinetic properties, which with creative expression including specific subcellular compartmental localization could allow for a variety of light wavelengths and channels to precisely tune neural responses. Although cation channels and anion pumps have been expressed and used to drive neuronal activity in rodent auditory brainstem neurons and auditory neocortex, the expression of these channels requires either the postfertilization transfer of the genetic material, typically using a viral vector, or transgenic techniques, neither of which is used in humans at this time. In addition, the channel kinetics of the available channels limit the rate of stimulation to around 50 to 60 Hz, much lower than the several hundred Hertz spiking that can been seen in SGNs as they follow the envelope of a sound stimulus.
Similar to optogenetics, thermogenetics uses a thermosensitive ion channel that has recently been described, which has been shown to allow for depolarization of neurons with the focal application of heat, such as IR light. Similar limitations to the expression of these channels as for optogenetic stimulation exist.
In addition to excitation of exogenously expressed thermosensitive channels, IR light has been shown to directly stimulate neurons. The mechanism underlying this is thought to be focal thermal changes in the plasma membrane capacitance, which results in depolarization. Shapiro and colleagues (2012) demonstrated that this excitation is due to a focal, reversible increase in temperature because of IR energy absorption by water. This increase in temperature results in a change in the local capacitance of the plasma membrane and leads to membrane depolarization. When compared with optogenetic and thermogenetic techniques, IR stimulation has the advantage of not requiring the expression of special ion channels or the infusion of special compounds. In a series of reports, Richter and colleagues demonstrated neural excitation with IR energy and defined the amount of energy required for this excitation, the temporal fidelity, and the spread of excitation from focal IR pulse, which was better than that of electrical stimulation. Littlefield and colleagues (2010) demonstrated that IR light could activate auditory nerve fibers by using IR light directed through the round window. However, several recent studies have challenged the underlying assumptions of how IR energy stimulates the auditory system. The rapid rise in temperature could result in pressure wave formation (up to about 60 dB sound pressure level equivalent), which could then stimulate remaining hair cells and drive an auditory response (optoacoustic effect). In addition, Verma and colleagues (2014) demonstrated that in a completely deafened cochlea, IR stimulation was unable to drive cochlear nucleus responses. There are numerous challenges to implementing these strategies. However, as water is the main molecule that absorbs IR energy, the light source needs to be very close to the target neuron because the surrounding fluids cause a significant decrement in the amount of energy available to drive neural responses. When compared with existing CI technology, the energy requirement for IR stimulation was far in excess. Further work needs to be performed to clarify the mechanisms of how IR energy directly stimulates central auditory pathways.
IR light can also be used to free caged compounds that can drive neural excitation. One example of this would be the use of light to break a photosensitive bond between glutamate and an inactivating caging compound.
With continued refinement, focused optical stimulation of the SGNs holds promise to overcome many of the technical and perceptual challenges currently present with modern CIs; this may take several forms but is mainly geared toward the creation of more functionally independent channels of information flow. It is also likely that novel coding and stimulation strategies will be needed for optical CIs to take advantage of the increased information channels and channel independence.
Intraneural cochlear implantation
Contemporary intrascalar CI electrode arrays are arguably the most successful neurosensory rehabilitation prosthesis, although as reviewed above, significant perceptual challenges remain including listening in noise, music perception, impaired pitch perception, and poor sound localization even with bilateral CIs. These deficits may be a result of the spread of excitation due to the high levels of current required to overcome the distance between the electrodes in the array and the excitable neural elements, the shunting of current away from these neural elements by the electroconductive perilymph, and the shielding effects of the modiolar bone covering the neural elements. Optical stimulation, as outlined above, is one mechanism that holds promise to refine the ability to precisely stimulate neural elements and thus reduce the spread of excitation and channel interaction. Another strategy is direct stimulation of the neural elements in the modiolus with intraneural electrodes. Intraneural implantation may offer several advantages over intrascalar electrode arrays including lower threshold currents because of direct interaction of the electrode and the neural elements (possibly allowing for an increased number of independent channels), the ability to access more apical fibers resulting in better stimulation of lower-characteristic-frequency neural elements, lower risk of facial nerve stimulation due to the electrode being farther from the facial nerve, and less anatomic limitations to implantation for dysplastic or ossified cochleas. Increased potential for neural injury because of insertional trauma represents a significant hurdle for stimulation with intraneural electrodes.
Djourno and colleagues (1957) were the first to chronically implant an electrical auditory prosthesis as described above. From 1964 through the middle of the 1980s, Simmons and colleagues published a series of reports investigating the direct intraoperative stimulation of the auditory nerve, implantation of an intraneural device into the auditory nerve, subsequent work in animal models, and a return to human direct nerve implantation. Amazingly, the initial human experiments resulted in auditory perceptions. Subsequent work in cats and then humans demonstrated that chronically implanted intraneural electrodes yield stable long-term thresholds and were well tolerated, although evidence of partial SGN loss and insertional neural trauma was found. As reviewed and cited in Arts and colleagues’ article (2003), the next development was that of the Michigan array, a series of electrodes on one or more thin shanks. In a series of experiments, these arrays were found to be well tolerated in both stimulating and nonstimulating conditions of the cochlear nerve and cochlear nuclei in animal models. When implanted in the modiolus, there was comparatively less cochlear and neural damage reported by Arts and colleagues than that reported by Simmons (1979). Both modiolar and intracanalicular auditory nerve implantations have been used. Investigators at the University of Utah have developed a multielectrode implant, termed the Utah electrode array (UEA) that uses a series of needle electrodes arranged in a square configuration that could be implanted into the modiolar nerve after a facial recess surgical approach. Badi and colleagues demonstrated that in cats implantation with variations of the UEA into the auditory nerve is feasible, seems to result in minimal histologic trauma to implanted nerves, and can elicit auditory responses. Lastly, Middlebrooks and Snyder (2007) implanted straight electrode arrays into the modiolar nerve and found that these electrodes could produce low current threshold, frequency-specific responses in the inferior colliculus central nucleus with less electrode interaction, and spread of excitation when compared with intrascalar electrodes. The studies by Simmons, those with the Michigan array by Arts and colleagues, those with the UEA by Badi and colleagues, and the work by Middlebrooks and Snyder (2007) all demonstrate that intraneural electrodes evoke auditory neural responses with less current levels than intrascalar electrodes. These studies demonstrate the feasibility of chronic intraneural implantation and stimulation and the possibility of increased numbers of independent channels with reduced channel interaction compared with current intrascalar electrode arrays. Furthermore, intraneural implants, if proven to be safe and at least not inferior to conventional intrascalar CIs, may offer a more reliable option for patients with malformed, brittle, or ossified cochleae.
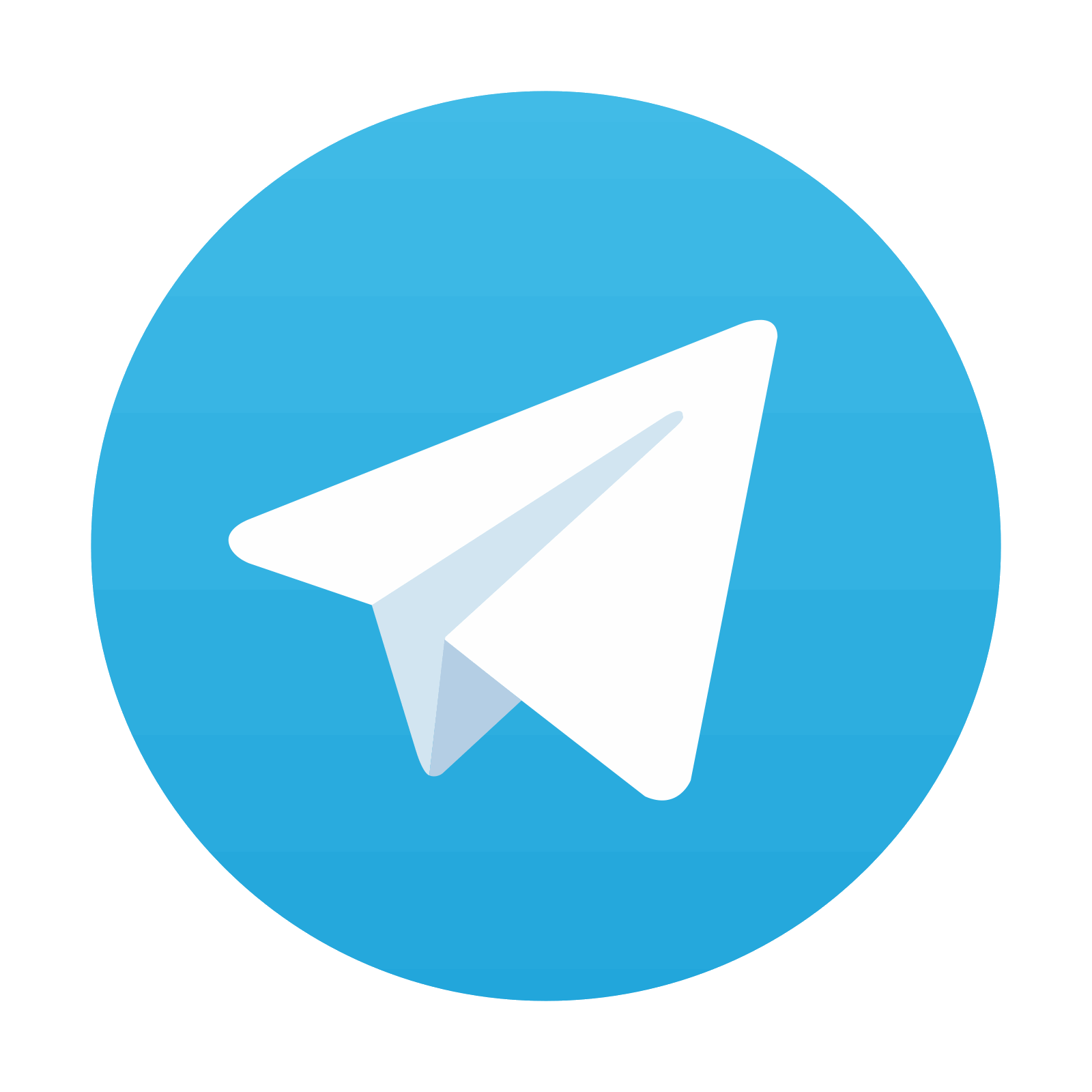
Stay updated, free articles. Join our Telegram channel
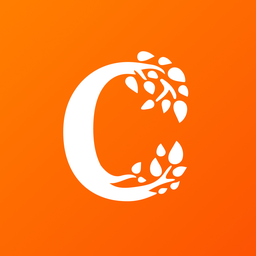
Full access? Get Clinical Tree
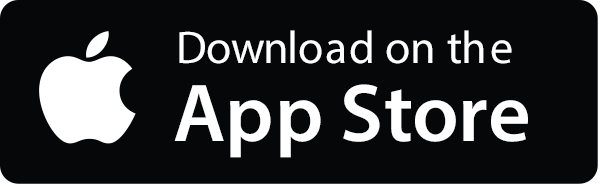
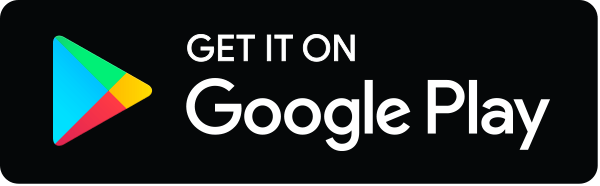