Fig. 2.1
Degree of Parkinson’s disease related degeneration in sleep-wake regulating nuclei as measured by cell counts. The healthy brain depicts the nuclei, and corresponding neurotransmitter or neuropeptide, known to regulate the sleep-wake cycle. The degree of neurodegeneration is depicted by increasingly lighter shading in the Parkinson’s disease brain. The percentage indicates the decreased number of neurons in Parkinson’s disease compared to controls
2.2.2 Acetylcholine
There are two primary cholinergic projection systems recognized to contribute to sleep-wake regulation: the basal forebrain and the pedunculopontine tegmental nucleus-lateral dorsal tegmental nucleus complex (PPN/LDT) in the brainstem. The primary cholinergic input to the cortex arises from the basal forebrain which includes three subpopulations: the medial septal nucleus, the diagonal band of Broca, and, in particular, the nucleus basalis of Meynert (NbM). In the brainstem, the PPN/LDT provide the major cholinergic input to the thalamus and the basal ganglia (including the SNpc) and send descending projections to the pontine reticular formation including the subcoeruleus, and the gigantocellular nucleus of the medulla [17, 25, 26].
The PPN/LDT neurons have either an equally high firing rate during both wake and REM sleep (Wake-ON) or they have significantly higher activity during REM than wake or non-REM sleep (REM-ON) [27]. Increases in activity occur 20–60 s in advance of state change [27]. This shift in firing rate prior to arousal demonstrates PPN/LDT’s critical role in driving the cortical desynchronization associated with wakefulness and REM sleep.
Dysfunction within the cholinergic system occurs early in the progression of PD and can be extensive. According to the Braak PD staging criteria, Lewy neurites begin to appear simultaneously in the basal forebrain and SNpc in stage 3, prior to dramatic cell loss in the SNpc [28]. Eventually, 32–46 % of the NbM neurons degenerate in PD [29, 30]. Degree of NbM loss does not correlate with disease duration [30], but in PD with dementia the magnitude can rival that observed in Alzheimer’s disease [29]. Mapping acetylcholinesterase activity in vivo with positron emission tomography indicates that the resultant decrease in cortical innervation is only 15 %; however, this can be more extensive than the deficit observed in Alzheimer’s patients (9.1 %) [31]. Postmortem examinations of parkinsonian brains indicate that 46 % of PPN neurons degenerate, particularly in the pars compacta component located in the caudal portion of the nucleus [32]. This degree of neuronal loss correlates with disease severity [32]. While positron emission tomography measures of AChE indicate that Alzheimer’s disease patients have no deficits in cholinergic thalamic innervation, there is a 12.8 % decrease in PD and a 19.8 % reduction in PD with dementia [33] indicative of PPN degeneration.
Deep brain stimulation (DBS) therapies reinforce the role that cholinergic brainstem nuclei play in sleep-wake regulation, and the impact that their degeneration plays in PD. Originally, the PPN was selected as a DBS target to address the postural instability and gait disturbances in PD [34]. Patients also report improvements in daytime sleepiness equivalent to a >50 % improvement on the Epworth Sleepiness Scale following bilateral PPN stimulation in comparison to STN alone [35]. Objective polysomnographic measures indicate increased sleep efficiency, a 70 % decrease in number of awakenings, and an almost twofold increase in time spent in REM sleep [35, 36]. The wake-promoting effects of PPN stimulation occur at lower stimulating frequencies (10–25 Hz), whereas high-frequency stimulation (80 Hz) can trigger sleep episodes [37]. Presumably, the higher frequency inhibits the wake-promoting thalamic projections from the PPN.
REM sleep behavioral disorder (RBD) diagnosis often precedes the clinical manifestation of PD and is considered an early manifestation of α-synucleinopathies including PD, dementia with Lewy bodies, and multiple system atrophy. Longitudinal studies of patients diagnosed with idiopathic RBD report that approximately 80 % of patients will subsequently be diagnosed with a parkinsonian disorder or dementia 12–14 years after the original onset of RBD [38, 39]. RBD is characterized by a loss of muscle atonia during REM sleep, which is traditionally maintained by a complex brainstem circuit including: PPN/LDT, locus coeruleus, subcoeruleus, medullary magnocellular reticular formation, and the ventrolateral reticulospinal tract (reviewed in [40]). Despite the strong comorbidity of RBD and PD, the severity of RBD symptoms is not correlated with impaired dopamine transporter binding as measured by positron emission tomography [41]. This suggests that the genuine pathology underlying RBD in the setting of parkinsonism involves degeneration of nondopaminergic circuits. Acetylcholine is an obvious candidate considering the early pathology in cholinergic circuits along with the prominent role of the PPN/LDT in the brainstem circuitry regulating atonia in rapid eye movement sleep. In PD patients, positron emission tomography reveals a significant correlation between the presence of RBD symptoms and decreased acetylcholinesterase in cortex and thalamus, but no significant relationship with either dopamine or serotonin measures [42]. Since these regions of cholinergic innervation have two different origins, the basal forebrain and the PPN/LDT, these results indicate concurrent degeneration of both pathways. PPN DBS does not reduce the increased muscle tone during REM observed in RBD patients [36]. Therefore, the decreased cholinergic innervation from the PPN is only part of the disrupted pathophysiology that underlies RBD in PD.
2.2.3 Hypocretin/Orexin
Owing to their axonal projection patterns [43] and their generally excitatory effects upon wake promoting neurons [44], the role of hypocretin/orexin (Hcrt/OX) neurons in regulating the sleep-wake cycle has long been appreciated [45, 46]. The Hcrt/OX-producing cell bodies are localized within the dorsomedial and lateral hypothalamus, including the perifornical area, and innervate histaminergic neurons in the nearby tuberomamillary nucleus and additional widespread ascending and descending projections to disparate brain regions [43]. Targets include the cortex, basal forebrain, amygdala, thalamus, SNpc, locus coeruleus, dorsal raphe, PPN/LDT, pontine reticular formation, gigantocellular nucleus of the medulla, and the spinal cord, in particular the intermediolateral cell column [43, 47]. Reciprocal innervation from these targets to the dorsomedial hypothalamus positions Hcrt/OX neurons to influence, and to be influenced by, circadian rhythms [48]. Regardless of which receptor they are binding (i.e., Hcrt1/OX1 or Hcrt2/Ox2), both Hcrt/OX peptides have excitatory downstream effects [45]. Additionally, many Hcrt/OX neurons have been found to corelease glutamate that may contribute to some of their effects on downstream targets [49].
In vivo electrophysiological investigations reveal that activation of the Hcrt/OX system is critical to orchestrating the transition between non-REM or REM sleep and wakefulness. Hcrt/OX neurons are most active during wake, particularly active waking, whereas their firing rate decreases during non-REM sleep and ceases during tonic REM sleep with a slight increase in activity during phasic REM [50, 51]. When applied experimentally, Hcrt/OX increases the firing rate of wake-promoting nuclei including: cholinergic neurons in the basal forebrain [52] and brainstem (LDT) [53], locus coeruleus [44], dorsal raphe [54], tuberomammillary nucleus [55], and the VTA [56]. Local application of Hcrt/OX into the basal forebrain [57], the locus coeruleus [58], or the LDT [59] increase wakefulness and decrease REM sleep. Optogenetic activation of the Hcrt/OX neurons triggers a transition from sleep to wakefulness, albeit with a delay between stimulation and arousal [60]. The interconnection of the multiple wake-promoting nuclei is emphasized by activation in downstream histaminergic neurons of the tuberomammillary nucleus and noradrenergic neurons of the locus coeruleus following optogenetic stimulation of the Hcrt/OX neurons [61]. Following sleep deprivation, sleep pressure overrides the effects of Hcrt/OX activation and the probability of arousal decreases and the downstream nuclei are not activated [61]. The increased firing rate of Hcrt/OX neurons throughout waking supports their hypothesized role in stabilizing the wake state, but occurrence of the highest firing rate during active wake may indicate additional roles in wake-related behaviors.
The number of Hcrt/OX neurons decreases by 23–62 % progressively over the course of PD [62]. Since the number of Hcrt/OX cells is dramatically reduced in narcolepsy [63], one might expect that the narcoleptic-like sleepiness observed in some PD patients (i.e., excessive daytime sleepiness and REM intrusions into daytime naps) would be explained by the loss of Hcrt/OX, along with the decreased dopaminergic signaling. Despite the dramatic degeneration of Hcrt/OX neurons, the level of hypocretin-1 in the CSF is not significantly reduced and does not correlate with sleep disruptions [64]. Experimental lesions in rats demonstrate that a 73 % decrease in the number of Hcrt/OX neurons is required for a concomitant 50 % reduction of Hcrt-1 in the CSF and increase in REM sleep [65]. Therefore, it seems that the remaining, viable Hcrt/OX neurons are able to compensate sufficiently to prevent significant decreases in CSF concentrations. However, the loss of excitatory drive on downstream wake-promoting nuclei still seems like a parsimonious explanation for some of the sleep-wake disturbances in PD patients. That being said, because of neuropathological heterogeneity and multiple additional factors (e.g., medications or comorbid disease) that influence sleep-wake state, it is difficult to pinpoint with certainty which parkinsonian-related sleep disturbance is attributable to the Hcrt/OX system degeneration.
2.2.4 Serotonin
Serotonin is produced in cell groups along the midline from the midbrain to the pons. The rostral potion of the serotonergic neurons in the pons and midbrain, including the dorsal raphe and median raphe, can be differentiated from the caudal portion based upon chemo- and cyto-architecture [66]. The neuroanatomical projections allow the dorsal and median raphe to be distinguished; although they project to similar areas, the terminal fields are distinct [67]. Targets of the dorsal raphe projections include the cortex, basal forebrain, thalamus, dorsomedial hypothalamus, SNpc, median raphe, locus coeruleus, PPN, subcoeruleus, and the sleep-promoting VLPO [24, 26, 68, 69]. Similarly, targets of the median raphe efferents include the cortex, basal forebrain, thalamus, dorsomedial hypothalamus, SCN, SNpc, VTA, locus coeruleus, dorsal raphe, PPN/LDT, and the subcoeruleus [26, 67].
Of the multiple serotonergic nuclei, the dorsal raphe primarily regulates sleep-wake cycles while the median raphe is integral to regulation of circadian rhythm [70–72]. The majority of serotonergic neurons within the dorsal raphe are type I, which demonstrate alterations in firing rate across behavioral state. Activity is highest during active waking, particularly when orienting to a stimulus, and decreases progressively across quiet waking and non-REM sleep [70, 72]. During REM sleep serotonergic neurons are silent, but resume wake-related levels of activity prior to the end of the episode [70, 72]. These findings indicate the dorsal raphe plays a key role in initiating the transition between stages.
In PD, decreased numbers of neurons are observed in both the dorsal raphe (37.2 %) [21] and the median raphe (56 %) [73]. Degeneration in each of these neurons may contribute separately to the sleep-wake disturbances observed in PD. Loss of the wake-promoting neurons in the dorsal raphe may contribute to the excessive sleepiness. Meanwhile, loss of circadian regulating median raphe neurons may contribute, in part, to the increased arousals at night and daytime sleepiness.
2.2.5 Norepinephrine
The locus coeruleus is the primary norepinephrine-producing nucleus in the brain. Targets of widespread noradrenergic efferents include the cortex, basal forebrain, thalamus, Hcrt/OX, dorsal raphe, PPN/LDT, subcoeruleus, and the sleep-promoting VLPO [26, 68, 74, 75]. Whether norepinephrine has excitatory or inhibitory influences on these downstream nuclei depends on the subtype of adrenergic receptors present. In the basal forebrain and dorsal raphe, excitatory effects are due to the presence of the α1- or β-adrenoceptors [76]. The VLPO and Hcrt/OX neurons express α2-adrenoceptors resulting in inhibitory responses [75]. In the PPN/LDT, the wake-ON neurons express α1-adrenoceptors and the REM-on neurons express α2-adrenoceptors allowing the effects of norepinephrine modulate state [77].
Activity of noradrenergic neurons is closely related to arousal. The neurons of the locus coeruleus are most active during wakefulness, decrease their activity during non-REM sleep, and are virtually silent during REM sleep [78]. Alterations in firing rate precede the EEG transitions to the corresponding arousal level [78]. Norepinephrine provides such a strong alerting signal that acute optogenetic stimulation results in immediate transition to wakefulness and long-term stimulation increases wakefulness and decreases non-REM sleep times [79]. Optogenetic inhibition of the locus coeruleus increases the number of sleep onsets and decreases the duration of wake episodes, but does not prolong the duration of sleep episodes [79]. However, arousals still occur during optogenetic inhibition of the locus coeruleus, indicating that this activity is not necessary and other components of the sleep-wake regulatory network can compensate in its absence [79].
In PD, 63–78 % of the neurons within the locus coeruleus degenerate [21, 80]. The extensive loss of this signaling pathway likely contributes to excessive daytime sleepiness in PD patients; the specific contributions, however, are difficult to disentangle from the effects of the degeneration in the other wake-promoting neurotransmitter systems.
2.2.6 Histamine
Histamine is produced in large cell bodies of the tuberomammillary nucleus located in the posterior hypothalamus. Widespread projections from histaminergic neurons innervate the cortex, thalamus, SNpc, VTA, locus coeruleus, dorsal raphe, LDT, pontine reticular formation, and the gigantocellular nucleus of the medulla [81]. The area of densest innervation is observed in the hypothalamus, including the Hcrt/OX cell bodies, and the basal forebrain [55, 81]. Afferents also target the sleep-promoting VLPO [68]. Four metabotropic histamine receptors have been identified (H1R-H4R); however, H4R is primarily expressed in the periphery. Signaling through both the H1R and H2R is excitatory, while H3R primarily functions as a presynaptic autoreceptor or heteroreceptor (reviewed in [82]).
Electrophysiological recordings of histaminergic neurons in freely moving mice across sleep-wake states reveal the highest firing rate during attentive or active wakefulness [83]. Activity decreases during quiet waking, ceases prior to the onset of sleep, and remains silent throughout non-REM and REM sleep [83]. Histaminergic neurons resume firing only when the animal is completely awakened, indicating that this signaling pathway is critical in the maintenance of wakefulness rather than initiating the transition from sleep to wake [83].
In contrast to all other wake-promoting nuclei, the histaminergic neurons of the tuberomammillary nucleus do not degenerate in PD [84]. In fact, the concentration of histamine is significantly increased in the SNpc (201 %) and other areas of the basal ganglia (159–234 %) [85]. In the SNpc, histaminergic innervation increases and the terminal fields are particularly dense surrounding remaining dopamine neurons [86]. This juxtaposition suggests that histamine may be important for promoting neuronal survival through stimulated release of interleukin-6 from astrocytes, which, in turn, triggers synthesis and secretion of nerve growth factor [86]. In the putamen, the concentration of the primary histamine metabolite tele-methylhistamine is the same in control and PD brains, indicating that the metabolism of histamine is unaltered despite the increased availability [85].
Pitolisant is an H3R antagonist/inverse agonist that is being developed to treat excessive daytime sleepiness. When administered to a parkinsonian feline MPTP model exhibiting daytime sleepiness, time spent awake is increased [87]. In clinical trials of PD patients, pitolisant reduced subjective sleepiness and resulted in a five-point decrease on the Epworth sleepiness scale [87].
It is difficult to know the role of increased histaminergic innervation of SNpc in general, let alone in the context of the disrupted sleep-wake cycle in PD considering the lack of information available regarding histaminergic innervation of other wake-promoting nuclei. However, the evidence indicating that histaminergic metabolism is preserved suggests that any loss of wake-promoting effect in histamine circuits would be due to degeneration of downstream targets.
2.3 Sleep-Promoting Circuit
2.3.1 Melanin-Concentrating Hormone
Melanin-concentrating hormone (MCH) neurons are intermixed with the Hcrt/OX neurons in the dorsomedial, lateral hypothalamus, but they are a distinct neuronal population [43]. Ascending and descending projections of the MCH neurons are extensive, but the relevant nuclei for sleep-wake regulation include: basal forebrain, thalamus, tuberomammillary nucleus, SNpc, VTA, PPN/LDT, dorsal and medial raphe, locus coeruleus, and the pontine reticular formation [88]. There are also reciprocal projections between the MCH neurons and the Hcrt/OX neurons [89]. MCH is an inhibitory neuropeptide, and >80 % of MCH neurons also corelease GABA [90] thereby increasing the potential inhibitory effects.
Evidence suggests that the primary role of the MCH neurons in sleep-wake regulation is to promote REM sleep. Electrophysiologically, the MCH neurons are maximally active during REM sleep and virtually silent during non-REM sleep and wakefulness [50]. Intracerebroventricular administration of MCH increases REM sleep amounts in a dose-dependent manner [91]. Optogenetic activation of MCH neurons during non-REM sleep increases the likelihood of transition to REM sleep, while activation during REM sleep increases the duration of the episode without altering the EEG power spectrum or muscle tone [90]. The extension of REM sleep duration is observed even in mice lacking the MCH receptor indicating that this effect may be mediated by the coreleased GABA rather than the MCH itself [90]. Due to the diffuse projections, it is conceivable that MCH promotion of REM sleep may occur through inhibitory projections to wake-promoting Hcrt/OX, histaminergic or basal forebrain cholinergic neurons, or by driving the REM-OFF neurons in the brainstem ARAS to cease firing. However, some evidence suggests that the MCH-histaminergic TMN or MCH-cholinergic basal forebrain circuits play key roles in mediating this effect [90].
In PD, the number of MCH neurons decreases by 74 % [62]. Loss of this signaling pathway may explain, at least in part, some of the nocturnal REM sleep deficits in PD patients. However, MCH is relatively unstudied in the context of PD and little is known about the specific impact of this deficit.
2.4 Conclusion
The neurodegeneration in PD extends beyond the hallmark nigrostriatal system and encompasses many other nuclei critical for the regulation of the sleep-wake cycle.
Due to the redundant projections and feedback loops, both negative and positive, throughout these nuclei, it is difficult to isolate the sleep-wake disruptions resulting from the loss of an individual neurotransmitter. This network must be considered to function as a unit and altered activity in one or more components impacts the output of the remaining members. The sleep-wake disruptions of PD patients reflect the integrated degeneration of multiple arousal related nuclei throughout the brain. While excessive daytime sleepiness and disrupted nocturnal sleep are inherent to the disorder itself, one cannot discount the secondary contributions of nocturnal movement disturbances or pharmacological therapies that may further worsen these symptoms.
References
1.
2.
3.
4.
Arnulf I, Konofal E, Merino-Andreu M, Houeto JL, Mesnage V, Welter ML, Lacomblez L, Golmard JL, Derenne JP, Agid Y. Parkinson’s disease a sleepiness: an integral part of PD. Neurology. 2002;58:1019–24.CrossRefPubMed
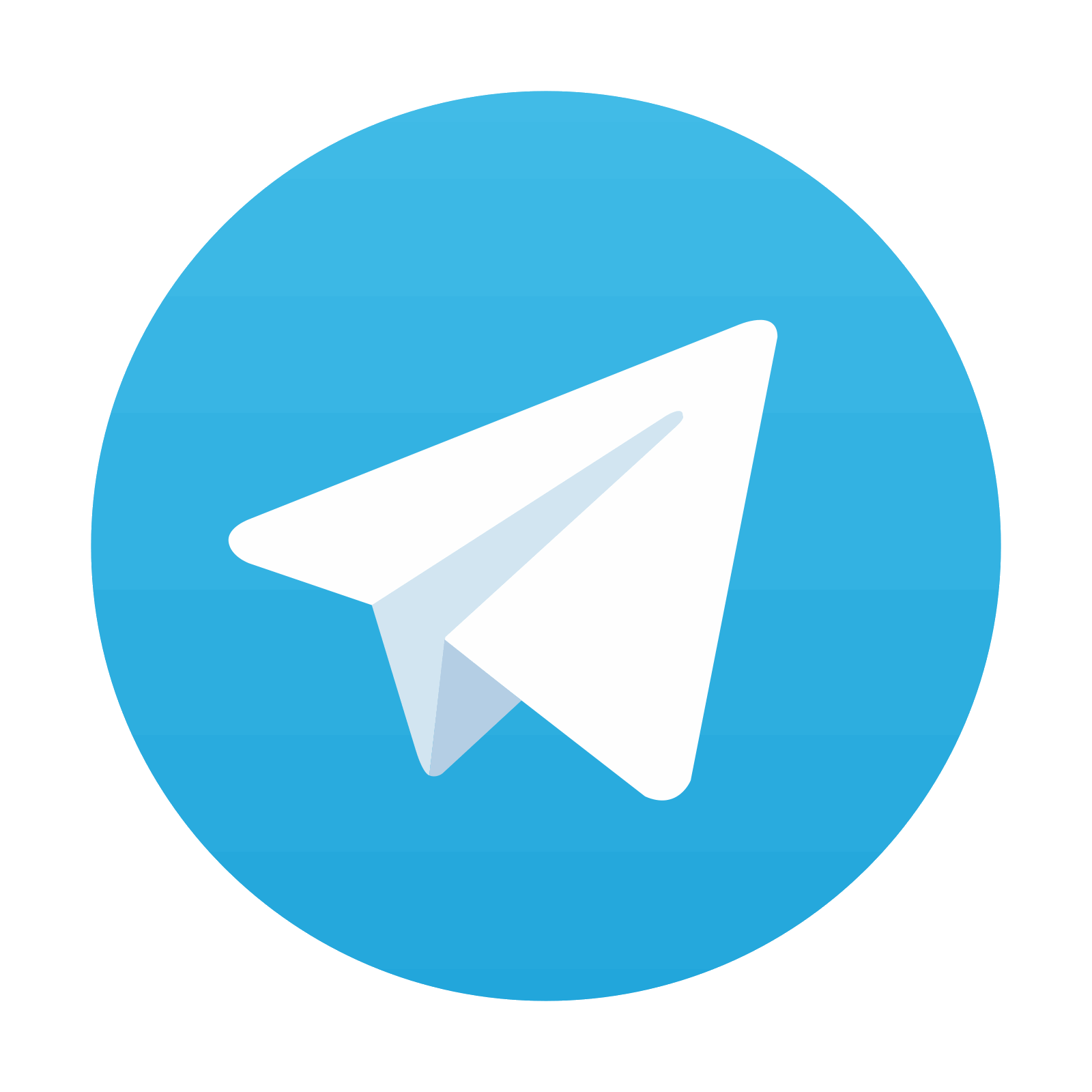
Stay updated, free articles. Join our Telegram channel
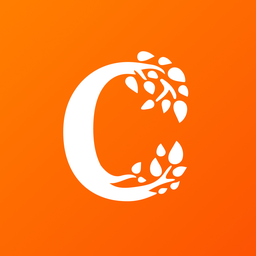
Full access? Get Clinical Tree
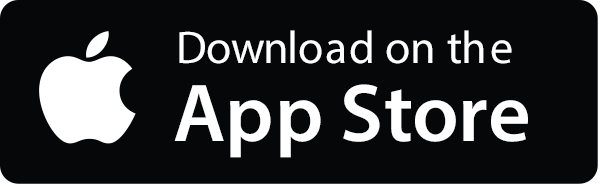
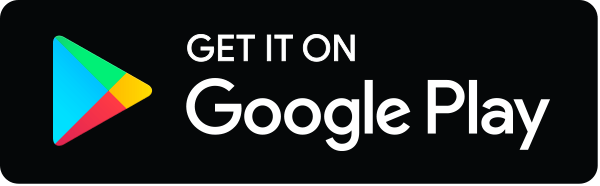