Lasers in Ophthalmology: Introduction
Ophthalmology was the first medical specialty to utilize laser energy in patient treatment, and it still accounts for more laser operations than any other specialty.
The main use of ophthalmic lasers was to treat various intraocular conditions. The transparency of the optical media allows laser light to be focused upon the intraocular structures without the need for endoscopy. Lasers are now used in many other areas of ophthalmic practice, including refractive surgery, cosmetic eyelid surgery, and diagnostic imaging of ocular structures.
Because laser surgery irreversibly changes tissue, ocular laser surgery should be performed only by ophthalmologists with laser experience.
The word “laser” is an acronym for light amplification by stimulated emission of radiation. Most sources of visible light radiate energy at different wavelengths (ie, different colors) and at random time intervals (noncoherent). The unique properties of laser energy are monochromaticity (single wavelength), spatial coherence, and high density of electrons. These allow focusing of laser beams to extremely small spots with very high-energy densities.
A laser consists of a transparent crystal rod (solid-state laser), or a gas- or liquid-filled cavity (gas or fluid laser) constructed with a fully reflective mirror at one end and a partially reflective mirror at the other. Surrounding the rod or cavity is an optical or electrical source of energy that will raise the energy level of the atoms within the rod or cavity to a high and unstable level, a process known as population inversion. When the excited atoms spontaneously decay back to a lower-energy level, their excess energy is released in the form of light. This light can be emitted in any direction. In a laser cavity, however, light emitted along the long axis of the cavity can bounce back and forth between the mirrors, setting up a standing wave that stimulates the remaining excited atoms to release their energy into the standing wave, producing an intense beam of light that exits the cavity through the partially reflective mirror. All of the light produced has the same wavelength (monochromatic) and phase (coherent), with little tendency to spread out (low divergence). The laser light energy can be emitted continuously or in pulses, which may have pulse durations of nanoseconds or less.
The principal lasers used in ophthalmic therapy are the thermal lasers, in which tissue pigments absorb the light and convert it into heat, thus raising the target tissue temperature high enough to coagulate and denature the cellular components.
These lasers are used for retinal photocoagulation; for treatment of diabetic retinopathy (Figure 23–1), retinal vein occlusions, and retinopathy of prematurity; for sealing of retinal holes; for photocoagulation of the trabecular meshwork, iris, and ciliary body in the treatment of glaucoma; and for the treatment of both benign (eg, choroidal hemangioma) and malignant (eg, choroidal melanoma and retinoblastoma) intraocular tumors.
These laser photocoagulators operate in continuous mode or very rapidly pulsed (thermal) mode. The green argon laser has been the standard but others include the krypton red laser; the solid-state diode laser, producing a near-infrared wavelength; the tunable dye laser, producing wavelengths from green to red; the frequency-doubled Nd:YAG laser, producing green light; and the thermal mode Nd:YAG laser, producing infrared light. The diode laser can be programmed to deliver very short pulses of laser energy (micropulse). Each pulse consists of a short “on” mode, to deliver the energy, and a longer “off” mode, allowing cooling of the target tissue. The PASCAL (PAtterned SCAnning Laser) is a frequency-doubled Nd:YAG laser that produces patterns of burns, such as a 5 × 5 square grid of 25 burns of 0.02-seconds duration, reducing the duration and discomfort of the extensive treatment required for panretinal photocoagulation (PRP) (see later in the chapter). Because laser light is monochromatic, selective absorption by specific wavelengths makes it possible to target-specific tissues while sparing adjacent tissues (Table 23–1). Absorption of laser light by specific tissues can be enhanced by intravenous injection of absorbing dyes such as fluorescein for short-wavelength laser or indocyanine green for long-wavelength laser.
Photodisruption lasers release a giant pulse of energy with a pulse duration of a few nanoseconds. When this pulse is focused to a 15–25 μm spot, so that the nearly instantaneous light pulse exceeds a critical level of energy density, “optical breakdown” occurs in which the temperature rises so high (about 10 000° K) that electrons are stripped from atoms, resulting in a physical state known as a plasma. This plasma expands with momentary pressures as high as 10 kilobars (150 000 psi), producing a cutting effect upon the ocular tissues. Because the initial plasma size is so small, it has little total energy and produces little effect away from the point of focus.
Photodisruptors are used for incision of posterior capsular thickening (posterior capsulotomy) or anterior capsular contraction following cataract surgery, peripheral laser iridotomy, and anterior laser vitreolysis. The principal laser of this class is the Q-switched neodymium:YAG laser.
The pulse duration of a femtosecond laser is even shorter, in the 10–15 second range. Produced by a solid-state neodymium:glass laser, the IntraLase is not absorbed by optically clear tissues. Thus, it is possible to focus it such as to produce precise cuts within the cornea, either as part of corneal refractive surgery or to assist in corneal dissection for penetrating or lamellar keratoplasty.
The photo-evaporation laser produces a long-wavelength infrared heat beam that is absorbed by water, and therefore will not enter the interior of the eye.
These lasers are used for evaporating away surface lesions such as lid tumors, bloodless incisions in skin or sclera, contact photo-incision and photocoagulation within the eye delivered through special probes, controlled superficial skin burns that can tighten the eyelid skin for cosmetic improvement, and correction of hyperopia by altering the corneal surface.
This class of laser includes the carbon dioxide, erbium, and holmium lasers.
Photodecomposition lasers produce very short-wavelength ultraviolet light that interacts with the chemical bonds of biologic materials, breaking the bonds and converting biologic polymers into small molecules that diffuse away. These lasers collectively are called excimer (“excited dimer”) lasers because the cavity contains two gases, such as argon and fluorine, which react into unstable molecules, which then emit the laser light. They are used in correcting refractive errors by precisely recontouring the cornea (photorefractive keratectomy [PRK], laser in situ keratomileusis [LASIK], laser epithelial keratomileusis [LASEK], and epithelial LASIK [Epi-LASIK]), removing superficial corneal opacities resulting from injuries or dystrophies, and treating recurrent corneal erosions (phototherapeutic keratectomy [PTK]).
The femtosecond laser is a focusable infrared (1053 nm) laser with femtosecond (10–15 seconds) pulses. By controlling the energy and firing pattern, tissue can be incised with minimal inflammation or collateral tissue damage, including very limited thermal damage to adjacent tissue. The cornea is transparent to the wavelength of the laser light so tissue can be precisely resected within the corneal substance. The femtosecond laser has been used for fashioning of stromal flaps for LASIK (IntraLASIK) and is being studied for other corneal procedures, including corneal grafting.
Therapeutic Applications of Lasers
In nonproliferative diabetic retinopathy, vision may be impaired by macular edema and exudates resulting from breakdown of the inner blood–retinal barriers at the level of the retinal capillary endothelium. Many patients with long-term diabetes mellitus will gradually develop diffuse obliteration of the retinal microcirculation, especially of the capillaries, resulting in generalized retinal ischemia. This ischemic state leads to neovascularization of the retina and iris, at least partly mediated by diffusible vasoproliferative factors released from the ischemic retina into the ocular fluids. Untreated retinal neovascularization leads to vitreous hemorrhages and traction retinal detachment. Iris neovascularization leading to neovascular glaucoma is rare unless the patient has had vitreoretinal surgery. (The clinical features of diabetic retinopathy are more fully discussed in Chapter 10.)
Diabetic macular edema can be treated by focal or grid pattern laser photocoagulation, which principally acts by augmenting the function of the retinal pigment epithelium, rather than by direct closure of microaneurysms as previously suggested. Burns 50–100 μm in diameter are applied, avoiding the foveal avascular zone, which is approximately 500 μm in diameter. The areas of leakage to be treated can be identified by clinical examination (zones of retinal thickening), by fluorescein angiography (areas of discrete or diffuse fluorescein leakage and areas of capillary nonperfusion associated with retinal thickening), or now usually by optical coherence tomography (OCT). Burn intensity (laser power setting) depends on the laser used. Using a shorter-wavelength laser (green or yellow), a slight change in color is required. Using a longer-wavelength laser (diode), the burn should be almost invisible. Diode micropulse and argon green lasers are equally effective at reducing the edema, but laser scars are 8 times more likely to be visible after argon green laser and there is tendency to better visual outcome with diode micropulse laser, with which theoretically there is reduced likelihood of progressive expansion of the area of laser damage.
The most effective treatment for retinal and iris neovascularization is PRP, which usually consists of treating the entire retina except for the area within the temporal vascular arcades, with burns 200–500 μm in diameter separated by 0.5–1 burn diameter (Figure 23–1). PRP requires a total of at least 2000 and sometimes 6000 or more burns, usually delivered over two or more sessions spaced 1–2 weeks apart. Retrobulbar, peribulbar, or sub-Tenon anesthesia is sometimes required, particularly if areas of the retina need to be treated again because of recalcitrant or recurrent neovascularization. Treatment is staged to reduce the incidence of uveitis, macular edema, exudative retinal detachment, and even shallowing of the anterior chamber with secondary angle closure. If there is significant macular edema, usually focal macular photocoagulation is performed before or together with PRP to avoid increase in edema. Intravitreal or orbital floor steroid (Triamcinolone) injection may prevent rebound macular edema after PRP. Currently it is restricted to patients requiring PRP and macular laser at the same time.
Adequate PRP is highly effective in producing regression of neovascularization. The exact mechanism of action has not been established, but reduction in the degree of retinal ischemia and production of diffusible vasostimulative substances are thought to be important. Reduction of ocular blood, suggesting reduction of oxygen demand in the retina, has been demonstrated after PRP. The type of laser used does not appear to influence the efficacy of PRP, but particular characteristics can be important in treatment, for example, the easier use of the diode infrared laser in the presence of vitreous hemorrhage and the more rapid laser delivery of the PASCAL.
PRP does not cause regression of the fibrosis associated with retinal neovascularization, which is responsible for tractional retinal detachment. Furthermore, PRP can be precluded by vitreous hemorrhage. Thus, PRP should be undertaken as soon as high-risk characteristics have developed. These include any new vessels on the disk with vitreous or preretinal hemorrhages, significant new vessels on the disk, and significant new vessels elsewhere with vitreous or preretinal hemorrhages.
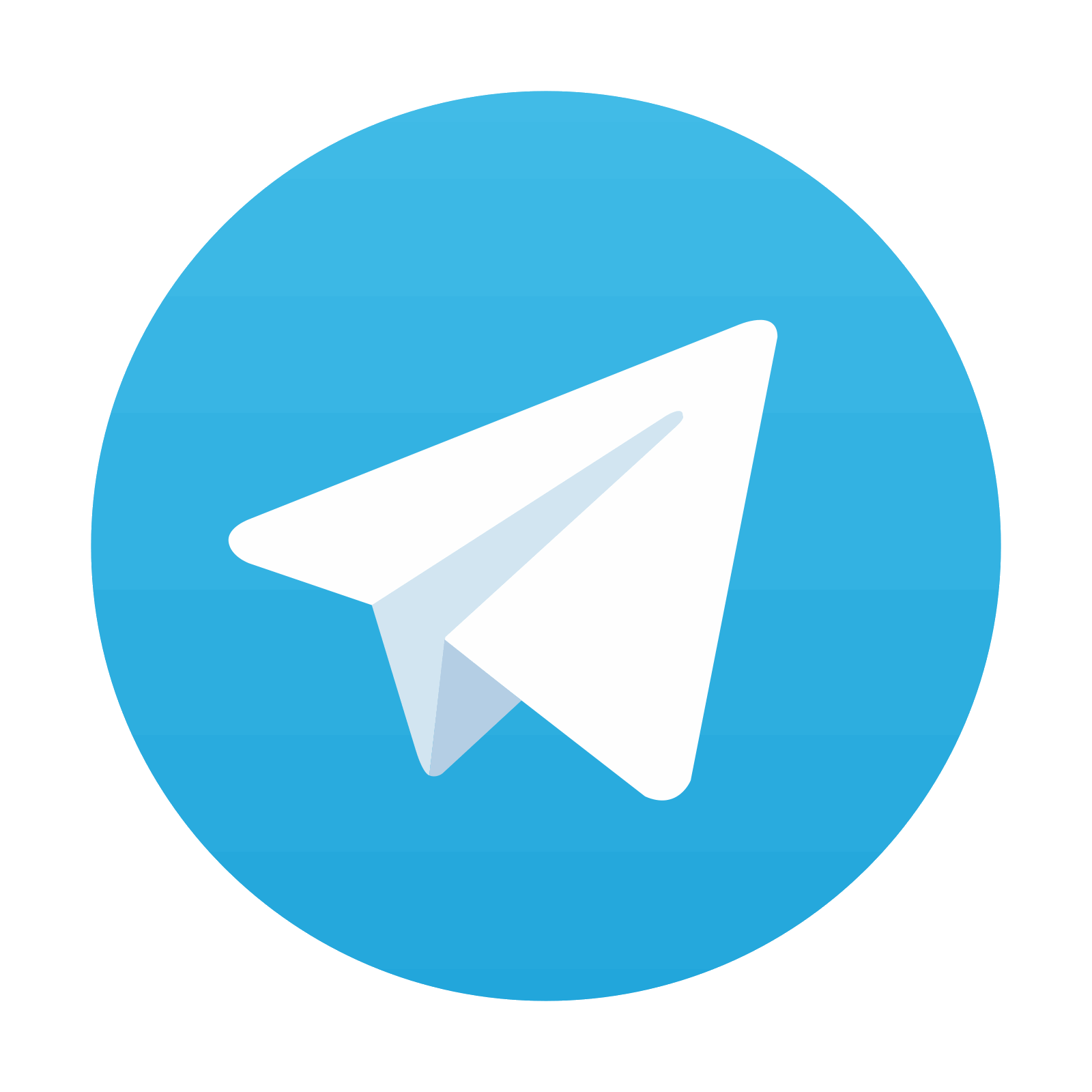
Stay updated, free articles. Join our Telegram channel
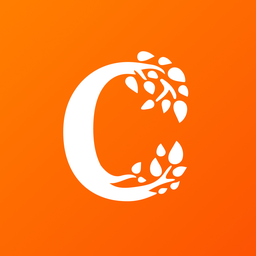
Full access? Get Clinical Tree
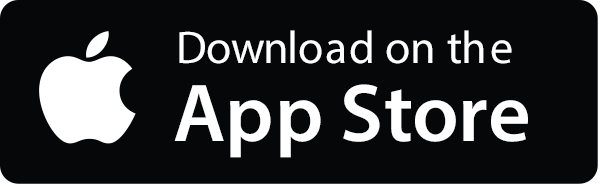
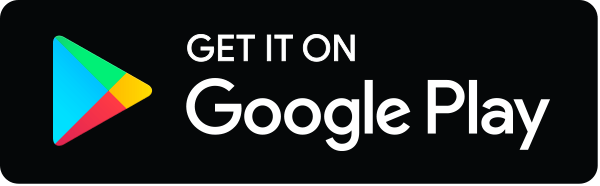