Genetic disorders are often thought of as interesting but rare. However, in a population of 100 000, there will be 10 children born with a genetic disorder, a further 10 who will develop genetic disorders in later life, and more than 10% of adults will have a chronic illness with a strong genetic basis. The advances in medical and molecular genetics have increased our understanding of disease processes, which can be illustrated particularly with examples of inherited ophthalmic disorders. Moreover, the increasing ability to individually, or within disease populations, define the genome, polymorphisms in gene expression, control of translation and the impact of environment on genetic expression or heritable as well non-heritable non-DNA influence on gene expression (epigenetics) has profoundly influenced our interrogation of disease pathogenesis and targeted therapeutic intervention.
Chromosomes and cell division
Chromosomes
Human cells contain 46 chromosomes (diploid), consisting of 22 almost identical pairs (homologous autosomes) and a pair of sex chromosomes, which constitute the karyotype of an individual. Chromosomes are numbered 1–22, as they decrease in size. The chromosome consists of two arms: the short arm is designated ‘p’ and the long arm ‘q’. During somatic division, or mitosis, each chromosome, and therefore gene, replicates precisely. Gamete formation involves a special reduction division (meiosis), in which the homologous chromosomes separate from each other so that each cell contains 23 chromosomes (haploid). Since chance determines which chromosome of a pair ends up in a particular gamete, there is a possibility of 2 23 combinations in the gametes. The different stages of the two forms of cell division are summarized below. The genes are located in a linear order along the chromosome, each gene having a precise location or locus. Each of a pair of chromosomes (homologous chromosomes) carries matching genetic information, i.e. they have the same sequence of gene loci. However, at any locus they may have slightly different forms, which are called alleles. Cytogenetic analysis of the chromosomes can be performed by looking at the banding pattern of individual chromosomes. Giemsa staining highlights the horizontal banding of the chromosome, by the characteristic pattern of light and dark staining (G-banding). More specific patterns of staining can be performed by looking at high-resolution banding with G-banding patterns at the early stages of mitosis (prophase; see below). Fragile sites are non-staining gaps that can be demonstrated only when the chromosomes are cultured in specific conditions (e.g. thymidine and folic acid deprivation) and then stained.
Cell division
Mitosis ( Box 3-1 )
During mitosis each chromosome, and with it every gene along its length, replicates to produce two identical daughter cells, so that every nucleated cell (except gametes) has 46 chromosomes (diploid). In culture, the cell cycle of most mammalian cells varies but is usually about 24 hours. Mitosis itself occupies approximately 1 hour of the total time, whereas the time taken to synthesize DNA required for replication is 6–8 hours ( Fig. 3-1 ). The gap phases G 1 , between mitosis and synthesis of DNA, and to a lesser extent G 2 , between synthesis and mitosis, govern the turnover of the cells because some cells can rest in G 1 for days. It appears that late in G 1 the cell passes a restriction point after which it will proceed through the rest of the cell cycle at a standard rate. During the S phase each chromosome replicates, each with its own individual pattern, although homologous pairs of chromosomes replicate synchronously. During G 2 , the cell gradually enlarges, doubling its total mass before the next mitosis. Some cells, however, do not divide once they are fully differentiated (e.g. neurones and erythrocytes) and are permanently arrested in a phase known as G 0 . Control of cell cycle is represented as a schematic in Box 3-2 .

Cyclins are regulatory subunits within the cell that are periodically synthesized and degraded, regulating cyclin-dependent kinases (CDK) essential for cell cycle control.
Cyclins (of which to date there are nine; A–I) bind to CDKs, forming an active complex that in a controlled fashion drives the cell through its stages via phosphorylation of unique protein substrates, such as retinoblastoma protein, Rb, allowing progression into the next phase of the cycle. Interaction of cyclin–CDK complexes with the retinoblastoma (Rb) protein family (Rb, p107, p130) is regulated by proteins that block cyclin–CDK activity (e.g. p21). p53, a cell cycle regulator, leads to increased p21 expression.

Meiosis: formation of gametes
Meiosis takes place in two stages, both having the same stages as mitosis. In the first stage of meiosis there is a prolonged prophase, in which cell division of only the chromosomes occurs so that each subsequent daughter nucleus contains half the number of chromosomes (23), resembling mitosis but in the haploid state. The prophase of the first meiotic division is complex and may be divided into a further five stages.
- •
Leptotene. Starts with the first appearance of the chromosome.
- •
Zygotene. The homologous chromosomes pair and bind closely to form bivalents.
- •
Pachytene. The main stage of chromosomal thickening; each chromosome consists of two chromatids and, because they are bivalent, has four strands. During this stage, chromatids of the chromosomes exchange material (crossovers), further ensuring a random assortment of paternal and maternal homologues.
- •
Diplotene. The bivalents start to separate (the centromere of each remains intact).
- •
Diakinesis. The bivalent chromosome formations separate and coil tightly.
Genetic makeup
The genetic makeup of each individual or of a specific patient population is a result of mutations and natural selection in the past. During cell division, the division of chromosomes may be imperfect. A mutation is a sudden change in the gross or fine structure of DNA, such as might be caused by a replication error or a crossover defect between misaligned chromosomes at meiosis, giving rise to deletion or duplication of DNA. The mechanisms by which chromosomal abnormalities may occur are discussed below. A mutation may affect a specific gene and thus the protein structure it codes for. Equally, the mutation may alter the structure of the protein in such a way that the function of the protein is changed only slightly but the metabolism remains unaffected. If the gene is destroyed altogether, including the nucleotide sequence, which is critically important for the protein that it encodes, then the individual will be at an immediate disadvantage. It is therefore not surprising that it is genetic diseases that occur as a result of harmful mutations, as opposed to diseases with multifactorial inheritance patterns, which have a predictable mode of inheritance.
Molecular genetics (DNA and genes)
The basis of inherited disease can be understood from information about chromosomal makeup, specific genes, and how proteins are encoded. The human haploid genome consists of 3 × 10 9 base pairs of double-stranded DNA (dsDNA). The genetic information that determines the sequence of amino acids in peptide chains is stored in the DNA by the order of the nucleotide bases: adenine (A), cytosine (C), guanine (G) and thymine (T). Three bases (a codon) code for a particular amino acid. A gene is part of the DNA that directs the sequencing of polypeptide chains. The gene itself consists of both coding regions ( exons ) and variable-length intervening regions ( introns ). There are also enhancer regions of the gene, which carry out regulatory activities, particularly the expression of genes in tissues. Similarly there is a family of regulatory molecules on the gene that activate or suppress both pairs of homologous genes ( Fig. 3-2 ).

How genes work: decoding DNA
All proteins are encoded in DNA and, as mentioned above, each amino acid is represented by a triplet code of bases (codon). Since the number of possible codons is greater than the number of amino acids, most amino acids, except methionine and tryptophan, may be encoded by more than one codon. The code AUG (methionine) acts as a starter signal for protein synthesis ( Box 3-3 ), and there are also codes that act as polypeptide chain terminators (stop codons).
Messenger RNA is produced from DNA transcription under control of the enzyme 5′-3′ RNA polymerase. Translation begins in the cell cytoplasm by the binding of mRNA to ribosomal subunits, to which an initiator tRNA molecule binds. Amino acid-specific tRNAs have anticodons specific for a triplet of nucleotides (codon), forming the aminoacyl-tRNA molecule, which binds to the A site on the ribosome under the control of an enzyme, aminoacyl-tRNA synthetase. Each amino acid is added to the C-terminal of the growing polypeptide chain, progressing from codon to codon in the 5′ to 3′ direction of the mRNA. The length of the polypeptide chain is regulated by specific start and stop codons.
Polypeptide chain synthesis occurs in two processes: transcription and translation ( Fig. 3-3 ). During transcription one strand of DNA forms a template for the synthesis of messenger RNA (mRNA), catalysed by the enzyme RNA polymerase. RNA polymerase binds to a specific DNA sequence known as the promoter , which signals the unwinding of the DNA helix, which acts as a template for the complementary base pairs. The process moves along the DNA molecule, extending the RNA molecule in the 5′ to 3′ direction. This process continues until it meets the termination signal on the DNA molecule. The first transcript is an exact replica of the single strand of DNA. Before leaving the nucleus the introns are excised and the exons are spliced together. On leaving the nucleus the mRNA acts as a template on cytoplasmic ribosomes for protein synthesis. The mRNA 5′ end is blocked before the whole molecule is transcribed with 7-methylguanine joined 5′ to 5′ and a 3′ poly-A tail, which aids transport into the cytoplasm. The poly-A tail is added after transcription by poly-A polymerase. Translation of nucleotide sequences is performed by specific transfer RNA (tRNA): amino acids, each specific for three nucleotide bases, are attached before polymerization into polypeptide chains. They are attached by their C-terminal ends and are transformed into high-energy molecules, from which peptide bonds form to produce polypeptides. Each tRNA (anticodons) is linked with the appropriate codons on the mRNA. This process of translation is controlled by a set of enzymes called aminoacyl-tRNA synthetases , which couple each amino acid to the appropriate tRNA molecule. The mRNA sequence is read three nucleotides at a time from the 5′ to 3′ direction along the mRNA. The polypeptide chain is constructed from its N-terminal end to its C-terminal end, and the growing C-terminal end of the protein remains activated by covalent attachment to the tRNA (peptidyl-tRNA molecule). The mRNA is coded with specific start and stop codons, which thus determine the length of the polypeptide chain. The initiation of polypeptide chain synthesis is under the control of initiation factor 2 (IF-2), which catalyses the reaction between a specific initiator tRNA molecule and the mRNA. The growing polypeptide chain must be exactly three nucleotides along the mRNA after the addition of each amino acid, which requires a multi-enzyme system incorporated within the ribosome. Ribosomes are half RNA by weight and have a groove that accommodates a growing polypeptide chain and a groove that accommodates each mRNA molecule. On the ribosome there are two different binding sites for tRNA. One site holds the tRNA and growing polypeptide chain, the peptidyl-tRNA-binding site (P site), and the other site holds the incoming tRNA molecule, the aminoacyl-tRNA-binding site (A site). The process of polypeptide chain elongation involves two steps. The first step involves an aminoacyl-tRNA molecule becoming bound to the A site adjacent to an occupied P site. The second step involves uncoupling the C-terminal end of the polypeptide chain from the tRNA in the P site and joining it to the tRNA molecule in the A site. The reaction is catalysed by peptidyl transferase , which is present on the ribosome. The new peptidyl-tRNA molecule is then transferred to the P site, releasing the now unoccupied tRNA molecule, and the whole process is repeated. The stop codon on the mRNA initiates a protein called release factor to bind to the A site, causing hydrolysation of the peptidyl-tRNA molecule on the P site and subsequent release of the polypeptide chain into the cell cytoplasm.

Genes are regulated by adjacent sequences on the genome. Upstream of the gene is the promoter , which is involved in the attachment of RNA polymerase to the DNA strand. Promoters have an element called a TATA box, which specifies the correct 5′ end of the RNA precursor. Several promoter-specific transcription factors have now been isolated, each specific for a gene promoter which, when bound, activates transcription. TATA-binding protein is a general transcription factor for RNA polymerases I, II and III. RNA polymerase III is responsible for the synthesis of a variety of small RNA molecules including tRNA and small subunits of ribosomal RNA. RNA polymerase II carries out the transcription of genes, and RNA polymerase I synthesizes the large subunits of ribosomal RNA.
Regulating gene expression is constantly active, has multiple pathways and is influenced by environment (epigenetics)
Ordering of DNA and accessibility for transcription
Histones are proteins that package DNA within nucleosomes. They act to form a structure that DNA spools around, are key to gene regulation and are influenced by environmental change ( epigenetics – changes in gene expression not caused by changes in DNA sequence ). Several families of histones exist and the formation and structure of the nucleosome, which in turn designates which areas of DNA are open for transcription, is influenced by key enzymatic modification, such as methylation, acetylation, phosphorylation, ubiquitination and SUMOlyation. For example, methylation of histone 3 at lysine residue 4 (H3K4Me3) at the promotor of active genes is associated with active transcription, as opposed to methylation at lysine 27, H3K27Me3, which results in gene repression.
Similar mechanisms may occur at DNA level. DNA methylation, in particular CpG repeats, confers an ‘off-switch’ where genes remain repressed. Methylation is essential for cell cycle and cell differentiation and alteration (which is part of epigenetic target) in methylation profile or sites of methylation can alter cell differentiation, fate and gene function.
Non-coding RNA and RNA-binding proteins constantly regulate gene expression
Gene expression can be further regulated during or after transcription (post-transcriptional regulation of gene expression). For example, encoded by nuclear DNA are microRNA (miRNA), which are small non-coding RNA molecules (around 22 nucleotides) that have complementary sequences with mRNA and usually result in regulation via gene silencing or via RNA-binding proteins, which are nuclear or cytoplasmic proteins that regulate RNA transcripts (for example within the spliceosome).
More work is still required on the understanding of RNA-binding proteins and biological and disease relevance; however, we do appreciate that miRNA have multiple mRNA targets, while each mRNA may also be targeted by several miRNA. Given that our genome codes for over a 1000 miRNA, there is therefore a rich network by which regulation of gene expression occurs, while aberrant expression of miRNA has been implicated in disease, in particular inflammation and cancer. Such miRNA has become very topical in the pathogenesis of degenerative disorders such as age-related macular degeneration. The formation of RISC (RNA-induced silencing complex) incorporates miRNA and activates enzyme RNA endonuclease III Dicer 1 that aids formation of small interference RNA (siRNA) within RISC and inhibition of gene expression ( Fig. 3-4 ).

Spliceosomes
When the gene is transcribed ( Fig. 3-3 ), the precursor mRNA is then further modified in spliceosomes where non-coding sequences are removed and coding sequences (exons) are ligated. Recognizing this process is important, as some proteins undergo ‘alternative’ splicing and thus form other active mRNA with the consequence of altered protein action and cell response and behaviour. Understanding mechanisms of splicing and the spliceosome therefore remain important, as altered activity is implicated in disease as well as targets for therapeutics.
Chromosome defects and gene mutations
During cell division the division of chromosomes may be imperfect. The extent, however, of genetically determined disease remains undetermined. The risk of genetic abnormality detectable at birth or in infancy is approximately 1 in 40. However, most fetuses with chromosomal aberrations are spontaneously aborted and it has been estimated that the frequency of genetic defects in live births is 0.6% and in stillbirths 5%. Chronic diseases with a significant genetic contribution occur in about 10% of the adult population. Abnormalities of the chromosomes are usually classified as numeric abnormalities, where somatic cells contain an abnormal number of normal chromosomes, or as structural abnormalities, where somatic cells contain one or more abnormal chromosomes, which in turn may affect either sex or autosomal chromosomes.
Numerical Chromosomal Abnormalities
Aneuploidy arises from failure of paired chromosomes to disjoin or as a result of delayed movement during anaphase, thus producing an extra copy of the chromosome (trisomy) or a missing copy of the chromosome (monosomy). Meiotic aneuploidy can occur during either of the stages of meiosis. Polyploidy describes a complete extra set of chromosomes (69 in triploidy), which usually results from fertilization of the ovum by two sperm cells. This usually results in miscarriage.
Autosomal Trisomies ( eFig. 3-1 )

Trisomy describes the individual chromosome that is somatically triplicated in the cell. Triplication of individual chromosomes gives rise to characteristic syndromes with multisystem disorders. Examples of autosomal trisomies include trisomy of chromosome 21 (Down syndrome), chromosome 18 (Edwards syndrome) and chromosome 13 (Patau syndrome). In each of the autosomal trisomies, mosaicisms (two or more cell lineages derived from a single zygote) may influence the clinical picture markedly, particularly when the normal cell line is present in a critical tissue. Trisomy, as mentioned above, may result from a failure of the separation of chromosome pairs during cell division (non-disjunction) or (in approximately 4% of cases) from translocation of one chromosome onto another inherited from one of the parents, thus giving the offspring a normal number of chromosomes but with a translocated copy of, for example, chromosome 21 (Robertsonian translocation).
Meiotic Aneuploidy
Similar chromosomal anomalies exist with the sex chromosomes, whereby an extra X or Y chromosome gives rise to a phenotype not distinguished in the normal population. Loss of an X chromosome gives rise to Turner syndrome (45 X0), which again may not present with the characteristic phenotype because of the prevalence of mosaic forms and therefore normal sexual development may be present. This is also true for Klinefelter syndrome, in which there is an extra X chromosome (47 XXY).
Structural chromosomal abnormalities
Structural chromosomal abnormalities result from chromosomal breakage. Normally single breaks are repaired quickly, but if more than one break occurs repair mechanisms may cause random rejoining of the wrong ends. Spontaneous breakage increases with exposure to mutagenic chemicals and ionizing radiation. The following structural abnormalities may occur:
- •
translocation – chromosomes break and exchange segments
- •
inversion – segment of chromosome is inverted in sequence
- •
deletion – section of chromosome is lost
- •
mutation – point mutation occurs with a change in a single base of a triplet code of a gene.
Gene mutations
Many types of mutation may occur throughout the alleles at each locus. Within the normal population, and in examples of inherited disease, mutations can occur that range from a single base pair to deletion of large gene segments involving many millions of base pairs. The description of these mutations has led to increasing availability of diagnostic and screening tools for genetic disease. Box 3-4 details the diversity of gene mutations that occur within the human genome.
Point Mutation (Nucleotide Substitution)
A single nucleotide change in the transcribed codon leading to a different amino acid substitution in the polypeptide chain – missense mutation.
A single nucleotide change which results in a stop codon, giving rise to a shortened polypeptide chain, or vice versa, resulting in an elongated polypeptide chain – nonsense mutation.
A single nucleotide base change which alters a critical splice junction and leads to abnormal RNA processing or a reduction in the normal gene product, or prevents the efficient addition of the poly-A chain for effective transcription – splice site mutation.
Deletions and Insertions (Frameshifts)
Deletion of one or two nucleotide bases leading to incorrect reading during translation and resulting in an inappropriate amino acid sequence and premature termination of the polypeptide chain.
Codon Deletions and Insertion (Repeat Expansions)
Insertion of a repeated codon (three nucleotide bases) that, as a consequence, interrupts the coding sequence. These mutations, known as triplet repeats , are found in myotonic dystrophy, the fragile X syndrome (X-linked mental retardation), Huntington’s disease and spinocerebellar ataxia.
Clinical genetics
There are several main types of genetic disorders: chromosomal (see above), mitochondrial, multifactorial, somatic cell genetic and single gene (autosomal and X-linked inheritance) disorders ( Box 3-5 ).
Autosomal Dominant
- •
Vertical transmission
- •
50% of offspring affected
- •
Males and females affected equally
- •
Variable expressivity
- •
Unaffected persons do not pass on trait
- •
Homozygotes are more severely affected
Autosomal Recessive
- •
Recessive gene does not cause disease in heterozygotes
- •
Disease expressed in homozygotes
- •
Males and females affected equally
- •
Constant expressivity
- •
Affected individuals have children who are carriers
X-Linked Recessive
- •
Vertical transmission
- •
Usually only males are affected
- •
All daughters of affected males are carriers
- •
Heterozygous females may be affected because of ‘lyonization’
- •
Variable expressivity
Autosomal inheritance
A trait that is determined by a gene on an autosome may be dominant or recessive. Heterozygotes are individuals with different alleles at the corresponding locus on the pair of homologous chromosomes. Homozygotes have the same allele. As such, autosomal dominant refers to the situation where a monogenetic disorder manifests clinically in the heterozygous state, and inheritance is usually from one parent only. Autosomal recessive traits refer to disorders that, while they can affect both sexes, require both abnormal genes for the disease to be clinically expressed.
Autosomal Dominant Disorders
The overall incidence of autosomal dominant inheritance ( eFig. 3-2A ) of disease in the UK is 7 per 1000 live births. Usually there is one affected parent, unless the trait occurs as a new mutation, in which case neither parent expresses the disease. Affected individuals have a 1 in 2 chance of passing it on to their child. This form of inheritance is not related to sex chromosomes and male-to-male transmission may occur. In contrast, X-linked disorders cannot be transmitted by males (see below). In clinical practice the detection of autosomal dominantly inherited disease is complicated by variation in expression of the gene and by new mutations. As such the clinical manifestation in an affected individual may not be apparent, giving rise to the view that the condition has skipped a generation. Most autosomal dominant disorders present clinically with features in later life, often after the carriers of the gene have completed their families. This raises difficulties in genetic counselling, and for this reason consideration is now being given to developing predictive tests based on gene tracking with DNA probes. The more severe the disorder the less likely the individual is to reproduce, so that the proportion of individuals affected as a result of new mutations will increase. Both new mutations and variable gene expression can combine to create difficult clinical decisions for the affected individuals as well as the clinical geneticist.

Autosomal Recessive Disorders
Autosomal recessive traits affect both sexes but the trait is manifest only if both abnormal genes are present ( eFig. 3-2B ), i.e. the patient has no normal allele at the affected locus. Usually both parents are heterozygous carriers of the gene in question and are clinically normal. Thus there is a 1 in 4 chance of any offspring being affected, a 1 in 2 chance of producing a heterozygous carrier, and a 1 in 4 chance of being normal. In rare recessive traits there is usually a strong family history of consanguinity between first cousins, who share 1 in 8 of their genes by virtue of their common ancestry. In practical terms the incidence of severe autosomal recessive disorders is low, making the risk to the sibling of first-cousin parents small. In the UK the overall incidence of autosomal recessive disorders is 2.5 per 1000 live births, one of the commonest being cystic fibrosis.
X-linked disorders
X-linked inheritance refers to the pattern of inheritance carried by the genes on the sex chromosomes. This inheritance therefore carries a characteristic family pedigree. An X-linked recessive trait carried on the X chromosome is manifest only in females when the homozygous state exists, because in heterozygotes the normal dominant gene would be expressed. Such individuals may express disease traits. Traits are thus transmitted from healthy female carriers or affected males. An affected male would pass the trait to all his daughters, who would then be heterozygous carriers, but he would be unable to pass the disorder on to his sons, who receive only his Y chromosome. In some X-linked recessive disorders a proportion of female heterozygotes are affected more as a trait. The explanation lies in the fact that only one of the X chromosomes in a cell is active (Lyon hypothesis; see Box 3-6 ). This inactivation occurs early in ontogeny and thereafter the descendants of the cell have the same inactive X chromosome. By chance, therefore, females may inactivate the healthy chromosome and thus manifest the disease.
Lyonization is the process of inactivation of one member of a pair of X chromosomes in every female somatic cell. The Barr body (densely stained mass of chromatin, which is the inactivated X chromosome) occurs in the trophoblast by day 12 after fertilization. Inactivation occurs only in somatic cells; it is random and then fixed for all subsequent generations of that cell line. The inactive X chromosome is not transcribed, except for a small region at the tip of the short arm. If loss of material occurs in one X chromosome, then the structurally abnormal chromosome is inactivated. About 30% of cells from buccal smears show a Barr body and 1–10% of female neutrophils will also show an inactivated X chromosome (drumstick). Females have random expression of either paternal or maternal X chromosome, which clinically gives rise to patchy expression of mutant X-linked genes in carrier females (e.g. retinitis pigmentosa and choroideraemia).
In the UK the incidence of X-linked inherited disorders is around 1 per 1000 live births. The term X-linked dominant has been used to describe X-linked inheritance where the heterozygous female is regularly affected. In these disorders males are frequently more severely affected and do not survive gestation (e.g. Aicardi syndrome: agenesis of the corpus callosum and chorioretinopathy). If the affected male does reproduce, none of the sons will be affected and all the daughters will be. Occasionally these pedigree patterns may be mimicked by autosomal traits that display sex limitation. If the affected males of an autosomal dominant trait with sex limitation are infertile, the pedigree pattern is identical to that of X-linked recessive traits, except that carrier females exhibit lyonization. The family pedigree will also show a smaller proportion of affected females than would be expected in X-linked dominant traits.
Mitochondrial inheritance
Cells have multiple copies of an extranuclear chromosome, contained in mitochondria. The mitochondria contain about 10 single circular chromosomes, which replicate independently of the nuclear genome. Within their genome there are the genes for tRNA and ribosomal RNA necessary for mitochondrial protein synthesis and peptides involved in cellular oxidative phosphorylation. As the acrosome of the sperm is lost during fertilization, the inheritance of mitochondrial chromosomes is exclusively through the female ova, which gives rise to a characteristic form of cytoplasmic inheritance with the following features: there is no transmission from males to their offspring, although both males and females can receive the defective gene from their mother; if all the mitochondria carried the gene, then all offspring of the affected woman would also be affected. However, the precise pattern of the disease may not be recognized if only a proportion of the mitochondrial genes are affected.
Multifactorial inheritance
There are many conditions that have a familial incidence, yet the incidence of affected siblings is less than can be accounted for by unifactorial Mendelian inheritance. This is because the clinical presentation is influenced by several genes and by environmental factors. In multifactorial inheritance both genetic and environmental factors combine in varying proportions in different individuals to permit the disease to be manifest. Each trait is determined by the interaction of a number of genes at different loci. Multifactorial inheritance may be continuous or discontinuous. In discontinuous multifactorial traits the risk within affected families is raised above that in the general population, but is low compared with that of single-gene defects. In continuous traits there is a range of disease expression, with individuals falling between two extremes (for example, blood pressure).
Multifactorial discontinuous traits have an increased incidence in affected families compared with that in the general population. If multifactorial inheritance is suspected, twin concordance and family correlation studies are undertaken. For instance, in multifactorial inheritance the incidence of the trait in monozygotic twins will exceed that in dizygotic twins. Some discontinuous multifactorial traits will also show unequal sex distribution, indicating that for one of the sexes the threshold for disease penetrance is higher than for the other sex, i.e. a higher proportion of underactive genes is required before the disease is expressed.
Disease associations
One approach to identifying disease associations with a specific genotype is to examine major genetic polymorphisms in a population and search for associations. Strong associations have been obtained between ankylosing spondylitis and HLA-B27 , and birdshot chorioretinopathy and HLA-A29. In practice it is perhaps the absence of such allelic correlations that is more useful in predicting disease occurrence. Disease associations can be expressed as relative risk by comparing the incidence of the allele within a control population with allelic expression in patients. Table 3-1 gives a list of some of the commoner associations. Currently, high-resolution typing that characterizes the nucleic acid sequence of major histocompatibility complex alleles has meant that, for example, classical DR4 associations are now further subtyped, e.g. the association of DRB1 * 0404 with sympathic ophthalmia and Vogt–Koyanagi–Harada syndrome.
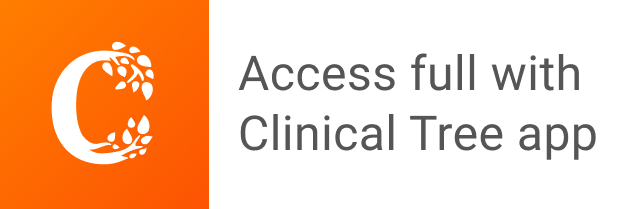