Fig. 8.1
The human evolutionary tree, indicating the temporal span of existence for different fossil hominin taxa. Each genus is represented by a different color. Species in genera other than Homo are generally referred to as “early hominins” by paleoanthropologists.
The earliest fossils tentatively considered to be members of the human family date to around 6–7 million years ago (Ma) (Senut et al. 2001; Brunet et al. 2002), which is close in time to the last common ancestor of humans and chimpanzees (Pan troglodytes) (Patterson et al. 2006). These fragmentary fossils are primarily identified as hominins, the group that includes living humans and fossil human species that arose after the split with chimpanzees, based on purported anatomical indicators of bipedalism (i.e., walking on two feet). The genus Australopithecus emerged around 4 Ma (Fig. 8.1) and is found in both eastern and southern Africa (White et al. 2006; Berger et al. 2010). Species attributed to this genus combine clear evidence of bipedalism with some degree of reduction in the canine teeth but retain many primitive features, including small brain size, curved fingers, and a projecting face. Evidence for tool use and manufacture in Australopithecus comes from the presence of a human-like elongated thumb in the hand skeleton (Alba et al. 2003), the recognition of cut marks on animal bones indicative of defleshing (McPherron et al. 2010), and the discovery of stone tools that date to around 3.3 Ma (Harmand et al. 2015), which predate the emergence of the genus Homo. A second genus of early human ancestor, Paranthropus, is also found in both eastern and southern Africa and can be distinguished from Australopithecus mainly by the presence of enlarged cheek teeth and robust cranial morphology to support more powerful chewing muscles (Constantino and Wood 2004). Species attributed to this genus are sometimes referred to as “robust australopithecines,” and taxa that are evolutionarily more primitive than the genus Homo, including both Australopithecus and Paranthropus, are generally referred to as “early hominins” by anthropologists.
All species of the genus Homo are generally considered tool users that consumed meat through either scavenging or hunting. The earliest fossils attributed to the genus Homo date to more than 2.5 Ma and are found in eastern Africa (Villmoare et al. 2015). In addition, a large fossil assemblage has been excavated in South Africa and attributed to the species Homo naledi (Fig. 8.1), which may represent an early member of the genus Homo (Berger et al. 2015). These fossils retain some primitive features seen in the early hominins, including a fairly small brain and curved fingers, but they also seem to depart toward H. sapiens in a number of aspects, including dental reduction, bipedal foot anatomy, and longer legs relative to arms. Subsequently, body size increased dramatically, with species such as Homo ergaster being similar in stature to H. sapiens but likely having a considerably heavier body mass (Ruff et al. 1997). These body proportions were largely maintained throughout the Pleistocene until the emergence of H. sapiens around 200 thousand years ago (Ka) (Arsuaga et al. 2015). Brain size increased during the course of evolution of the genus Homo, and after about 600 Ka some fossil specimens show brain sizes similar to H. sapiens (Ruff et al. 1997).
In Europe, the earliest hominin fossils date to around 1.2–1.4 Ma and are attributed to the species Homo antecessor (Bermúdez de Castro et al. 1997; Carbonell et al. 2008). The earliest fossils that show clear Neandertal features date to somewhat later, around 500 Ka, and are found at the site of the Sima de los Huesos (SH) in the Sierra de Atapuerca in northern Spain (Arsuaga et al. 2014). The Neandertals, Homo neanderthalensis, emerged sometime around 200 Ka (Fig. 8.1) and show a considerably enlarged brain and perhaps some evidence of cold adaptation in their limb proportions (Harvati 2007). Neandertals survived in Europe until around 40 Ka and were adept hunters that buried their dead and had a controlled use of fire. Some disputed evidence of symbolism, in the form of personal adornments and engravings on cave walls, has been found at a few Neandertal sites (Caron et al. 2011; Rodríguez-Vidal et al. 2014). While the Neandertals were not ancestral to H. sapiens, some evidence of hybridization has been found (Fu et al. 2015).
H. sapiens emerged in Africa around 200 Ka, and the earliest H. sapiens fossils have been found in eastern Africa (White et al. 2003; McDougall et al. 2005). While early H. sapiens fossils are also found at two sites in the Middle East, dating to around 100 Ka, H. sapiens did not enter Europe until much later (around 40 Ka). Evidence of symbolic expression is present at a few sites in southern Africa in the form of personal adornments around 70–80 Ka (Henshilwood et al. 2004) and in Europe around 35–40 Ka in the form of cave paintings (Pike et al. 2012).
The evolution of language is a long-standing controversial topic in evolutionary studies, in part because language is often considered a defining feature of what it means to be human. Nevertheless, the study of language in fossil specimens has been a frustrating endeavor, partly due to the fragmentary nature of the fossil record but even more due to the difficulty in establishing a correspondence between anatomical features of the skull and skeleton and language capacities.
8.2 Language Studies in Hominin Fossils
Given the degree of neural processing involved in producing and perceiving auditory stimuli and decoding symbolic signals (Ramsier and Rauschecker, Chap. 3), brain morphology represents an obvious first approach to studying language evolution. Some attempts have been made to identify the development of language centers, in particular Broca’s area, in fossil hominin endocasts (fossilized impressions of the brain surface) (Tobias 1987; Holloway et al. 2004). These studies have generally concluded that language capacities were not present in the early hominin genera Australopithecus and Paranthropus and that language capacity first appeared during the evolution of the genus Homo. However, drawing clear functional inferences from the gross morphology and contours of the brain surface is problematic (Sherwood et al. 2003), and it is well-known that linguistic processing in the brain is not restricted to Broca’s area (Grodzinksy 2000).
Because of its direct role in the production of vocalizations, the anatomy of the supralaryngeal vocal tract (SVT) has been considered fundamental by many researchers for establishing the presence of spoken language in fossil hominins, particularly Neandertals (Laitman et al. 1979). The SVT in mammals is composed of a horizontal segment (the oral cavity or mouth) and a vertical segment (the pharynx or throat). In order to produce the quantal vowels /a/, /i/, and /u/, the two segments of the SVT should be approximately the same length (i.e., a 1:1 ratio). In adult H. sapiens, both segments are of a similar length due to the combination of a short oral cavity and a low position of the larynx in the neck. This latter feature, often called “the descent of the larynx,” is considered to be exclusive to H. sapiens and a direct adaptation for the production of spoken language (Lieberman et al. 1992).
In contrast, Lieberman (2007a) argued that Neandertals depart from a 1:1 ratio for the two segments of the SVT due to the combination of a shorter vertical segment, implying a higher placement for the larynx, and a longer horizontal segment related to a higher degree of facial projection. While the Neandertal ratio likely does depart from the 1:1 ratio in adult H. sapiens, it is similar to that of a ten-year-old H. sapiens child who is capable of producing the quantal vowels (Boë et al. 2007).
Other researchers used a prediction model for larynx height based on correlations with various dimensions of the skull and mandible in H. sapiens (Boë et al. 2002) and found Neandertals were characterized by a low-placed larynx. In fact, a low-placed larynx is not an exclusively H. sapiens feature since it is also found in other mammals in which it is related to biological functions other than speech production (Fitch and Reby 2001). Furthermore, Nishimura et al. (2006) have shown that the larynx in chimpanzees descends during the growth process. Although its final placement is not as low as in humans, chimpanzees appear to represent an initial stage in the descent of the larynx, and this feature was likely already present in the last common ancestor of chimpanzees and humans.
Another line of evidence into reconstructing the SVT in human fossils is the study of the morphology and dimensions of the hyoid bone, which has insertions for the muscles of the tongue and of the pharynx. The H. sapiens hyoid bone differs from chimpanzees in which the body of the hyoid bone is expanded and box shaped and houses an extension of the laryngeal air sacs on the ventral face. In contrast, the hyoid body in H. sapiens is not expanded, takes on a horseshoe shape, and lacks laryngeal air sacs (Aiello and Dean 1990). The absence of laryngeal air sacs is an important anatomical feature for creating subtle, timed, and distinct sounds, which are necessary for human speech.
The hyoid is among the rarest bones preserved in the hominin fossil record. The single Australopithecus afarensis specimen shows a hyoid body with morphology and dimensions similar to those of chimpanzees (Alemseged et al. 2006), while the hyoid in Neandertals and the Atapuerca (SH) hominins is human-like (Arensburg et al. 1989; Martínez et al. 2008). This suggests that laryngeal air sacs were still retained in A. afarensis but were absent in Neandertals and the Atapuerca (SH) hominins (de Boer 2012). Thus, although the vocal tract proportions and their functional significance in Neandertals continue to be a subject of debate (due in no small part to the amount of soft-tissue reconstruction necessary), it seems likely that middle (780–128 Ka) and late Pleistocene (128–10 Ka) hominins possessed the capacity for speech production.
Ancient DNA analysis also offers some insights into the evolution of human language. Neandertals share with H. sapiens two derived mutations in the FOXP2 gene that are absent in chimpanzees (Krause et al. 2007). Although the precise functional implications of these two derived mutations for language ability are not well-understood, certain mutations in this gene are known to produce speech and language disorders in living humans (Lai et al. 2001). These results offer tantalizing evidence for the possible presence of spoken language in Neandertals.
In contrast, there is a movement to limit language capacities solely to H. sapiens based on evidence for symbolic behavior in the archaeological record. Several researchers argue that symbolic objects and activities such as cave paintings, “Venus” figurines, grave goods, and use of body ornaments and/or pigmentation should be taken as clear and direct indicators of symbolic mental representations and language (Henshilwood et al. 2001; Tattersall 2009). Indeed, the emergence of these symbolic behaviors in the archaeological record has been explicitly linked with the beginning of human spoken language (Tattersall 2004). Such evidence for symbolic activities has historically focused on European Upper Paleolithic sites, which are relatively late in time (generally younger than 40 Ka), and has been attributed to H. sapiens. Earlier evidence for body ornaments and abstract engravings has been documented at a few sites in northern and southern Africa, dating to approximately 80 Ka (Henshilwood et al. 2002; Bouzouggar et al. 2007). Although no diagnostic skeletal remains have been recovered from these sites, it is generally held that the activities were carried out by H. sapiens individuals. Evidence for Neandertal symbolic behavior is more limited (Jaubert et al. 2016), coming mainly from a few sites in western Europe, and remains controversial (d’Errico et al. 1998; Mellars 2010). While symbolic activities in the archaeological record likely do reflect the presence of human spoken language, there is no reason to suspect that the absence of archaeologically detectable symbolic behaviors precludes the possibility of some form of spoken language.
This review suggests that the balance of evidence based on the preserved anatomical features of the skeleton, as well as ancient DNA, is most consistent with some speech production capability characterizing the human evolutionary lineage prior to the emergence of H. sapiens. With this in mind, the chapter reviews research into the evolution of audition in fossil hominins and discusses the potential of this avenue of inquiry for providing new insights into this topic.
8.3 Hearing in Living Primates
The auditory capacities of numerous primate species have been measured in the laboratory by a variety of techniques (Ramsier and Rauschecker, Chap. 3). A review of primate hearing has stressed the fundamentally mammalian nature of the audiograms reported among primate species, including humans, in terms of high-frequency cutoff and low-frequency sensitivity, as well as sound localization abilities (Heffner 2004). However, a detailed study of primate audiograms does reveal some important distinctions between the major subgroups within the primate order (Coleman 2009).
The majority of Old World monkey species are characterized by a W-shaped audiogram with two peaks of heightened sensitivity, often at around 1 and 8 kHz, and a region of reduced sensitivity in the midrange frequencies, usually around 4 kHz (Coleman 2009). Despite some variation in a few species (Owren et al. 1988), the widespread distribution of a W-shaped audiogram among Old World monkeys suggests that this represents the primitive condition from which the human audiogram evolved.
Hearing in chimpanzees was first studied by Elder (1934) and subsequently studied by Kojima (1990). The results of these two studies coincide in showing a W-shaped audiogram with two peaks in heightened sensitivity at 1–2 kHz and 8 kHz and a relative reduction in sensitivity at around 4 kHz (Fig. 8.2). In addition, the high-frequency cutoff was around 33 kHz (Elder 1935), which is substantially higher than in humans but lower than in other primate species tested subsequently.


Fig. 8.2
Behavioral audiograms of humans and two chimpanzees (Pan troglodytes: 1, 2). The solid line for each species/individual represents the minimum sound level at a given frequency that a subject can perceive correctly at least 50% of the time. Points lower on the curve indicate a greater auditory sensitivity, and any sounds that fall above the curve are audible. Humans show a U-shaped audiogram with a broad region of heightened sensitivity in the midrange frequencies and a best frequency around 3 kHz. Chimpanzees show a W-shaped audiogram with two points of maximum sensitivity around 1 and 8 kHz and a loss in sensitivity around 4 kHz. The human audiogram presented here corresponds to the M. A. P. function from Sivian and White (1933, p. 313), and the chimpanzee data are from Kojima (1990).
The H. sapiens audiogram generated under free-field conditions (Fig. 8.2) suggests good low-frequency sensitivity (below 1 kHz) compared to that in most primate species and a broad range of heightened sensitivity in the midrange frequencies (1–4 kHz) with the best sensitivity around 3 kHz (Sivian and White 1933). This is followed by a subsequent decrease in sensitivity to higher frequencies with the lowest high-frequency cutoff of any primate species (Heffner 2004). Although there is some variation in the published human audiograms, in non-hearing-impaired people, the best sensitivity is consistently between 2 and 4 kHz and the high-frequency limit is around 20 kHz.
The greatest contrast between the chimpanzee and human audiograms, then, is in the midrange frequencies where humans appear to have widened the region of maximum sensitivity. Despite assertions to the contrary (Heffner 2004), the human audiogram appears to be unique and evolutionarily derived (i.e., differing from the ancestral condition) within the living primates, suggesting the possibility of an auditory specialization. Given this difference in the audiogram between chimpanzees and humans, the study of auditory capacities in fossil hominins has the potential to reveal when the H. sapiens auditory pattern first emerged during evolutionary history. The region of heightened auditory sensitivity in humans also coincides with a portion of the frequency range of spoken language, which reaches up to around 6 kHz (Fig. 8.3). Although the majority of the sound power of vowels is concentrated below about 2.5 kHz, many consonants are concentrated at the higher frequencies (Hughes and Halle 1956). Thus, the appearance of the human auditory pattern also may have implications for communicative capacities.


Fig. 8.3
The human audiogram and speech frequencies. The frequency range of conversation-level human spoken language, the “speech banana,” is indicated by the shaded region. The region of heightened sensitivity in the human audiogram overlaps with a portion of the frequency range of sounds emitted during spoken language. (Reprinted from Quam et al. 2012; used with permission from Nova Science Publishers, Inc.)
8.4 Studying Hearing in Fossils
The appearance of the ossicular chain (malleus, incus, and stapes) of the mammalian middle ear had clear auditory consequences, most notably the emergence of high-frequency hearing in early mammalian forms, including primates (Masterton et al. 1969; Rosowski and Graybeal 1991). The functional implications of size and shape variation in ear anatomy underlie much of modern audiological research and have made it possible to predict aspects of the known hearing capacities in living primate species from their ear dimensions (Coleman and Ross 2004; Coleman and Colbert 2010) (Nummela, Chap. 2). There are clear anatomical differences in the outer, middle, and inner ears between humans and chimpanzees (House 1972; Quam 2006), and auditory differences also have been reported (Sect. 8.3). The correlations between structure and function established in these studies can be used to predict aspects of the hearing capacities in fossil taxa (Coleman et al. 2010).
Comparative genomic studies have revealed changes during the course of human evolutionary history in several genes related to the development of the auditory structures (Clark et al. 2003) and hearing (Scally et al. 2012). Importantly, both the functional morphology (Rosowski 1994) and developmental biology of the ear have been studied across mammals, including humans (Frenz et al. 2001; Mallo 2003). The majority of the structures of the human middle and inner ears are already formed at birth, and their development is under tight genetic control. In evolutionary terms, the middle and inner ears seem to be among the most conservative anatomical regions of the body. Thus, a comprehensive study of the ear region in fossil hominins has the potential to shed new light on the evolution of sensory capacities in the human lineage (Quam et al. 2012).
8.4.1 Evolutionary Anatomy of the Ear in Fossil Hominins
Some anatomical differences from living humans in the outer and middle ears have been reported in the early hominins (Rak and Clarke 1979; Quam et al. 2015). Specifically, both A. africanus and P. robustus are characterized by an external auditory canal that is intermediate in length between humans and chimpanzees. At the same time, the cross-sectional area of the ear canal is enlarged and human-like in both early hominin taxa. The tympanic membrane in the early hominins is reduced compared with that in chimpanzees and resembles humans in absolute size. The tympanic cavity in P. robustus is similar in size to the mean size in both the chimpanzee and human, but the tympanic cavity in A. africanus is somewhat smaller (Quam et al. 2015). The mastoid region in chimpanzees shows a more extensive network of air-filled cavities (i.e., pneumatization) than in H. sapiens (Sherwood 1999), but both species show considerable variation, which makes it difficult to interpret the possible significance of differences in fossil hominin taxa.
The outer and middle ears in the genus Homo subsequently changed in several aspects compared with those in the early hominins (Martínez et al. 2004; Quam 2006). In particular, fossil specimens attributed to the genus Homo are characterized by a mediolaterally shortened ear canal, resembling H. sapiens. The tympanic membrane is enlarged and more similar in size to the larger tympanic membranes in chimpanzees than to the smaller values in H. sapiens. The volumes of the tympanic cavity and mastoid air cells are also enlarged in the genus Homo. All of these features are present in the Middle Pleistocene hominins from the Atapuerca (SH) site (Martínez et al. 2013) and also appear to characterize Neandertals (Quam 2006; Balzeau and Radovčić 2008). Although the data are more limited, some of these features also seem to be present in H. erectus fossils from Asia (Balzeau and Grimaud-Hervé 2006) and at least one early Pleistocene Homo fossil (SK 847) from South Africa; thus, they appear to have emerged very early in the evolution of the genus Homo.
Regarding the ear ossicles, the malleus in both A. africanus and P. robustus is derived and human-like in its proportions, and it is clearly distinct from chimpanzees (Quam et al. 2013b). While no incus is known for A. africanus, P. robustus shows a unique combination of a human-like malleus and ape-like incus. The resulting malleus/incus lever ratio in P. robustus is intermediate between the higher values in chimpanzees and the lower values in humans. The stapes in A. africanus resembles the chimpanzee stapes in its overall size, including the small size of the footplate (Moggi-Cecchi and Collard 2002). Although a different study suggested larger stapes footplates in early hominins (Braga et al. 2015), the discovery of additional stapes from both A. africanus and P. robustus has confirmed their small dimensions (Quam et al. 2013b). Thus, the early hominins seem to be characterized by a human-like malleus, while the incus and stapes are primitive and most similar to chimpanzees in their size and shape.
The ear ossicles in fossils attributed to the genus Homo show some changes from the early hominins but also preserve some primitive features. Specifically, the malleus/incus lever ratio in the Middle Pleistocene Atapuerca (SH) specimens is similar to H. sapiens (Martínez et al. 2004), indicating that a lengthening of the incus long process occurred. At the same time, the stapes remains small in overall size, including the footplate (Quam et al. 2006). The few known Neandertal ear ossicles show similar morphology and dimensions as in the Atapuerca (SH) specimens, while H. sapiens differs mainly in showing a larger stapes, including the footplate (Quam and Rak 2008; Quam et al. 2013a).
Studies of the inner ear in early hominin taxa have provided insights into their taxonomic relationships and hominin locomotion (Spoor et al. 1994). Although most analyses have focused on the semicircular canals, the cochlear basal turn is similar in size in chimpanzees and early hominins but slightly larger in humans. Nevertheless, little inference regarding hearing abilities can be drawn from these limited data. Perhaps more relevant, the length of the cochlea along the outer surface has been measured in several early hominin specimens and is shorter than in H. sapiens (Braga et al. 2015). If this is taken as a proxy measure for the length of the bony spiral lamina, it may indicate a shorter basilar membrane length in the early hominins. This shorter length would be consistent with a higher high-frequency cutoff (West 1985) above the approximate 20 kHz cutoff in humans, perhaps more closely resembling chimpanzees (about 30 kHz cutoff).
The cochlea in the genus Homo is generally larger than in the early hominins. The radius of the basal turn is similar in most members of the genus Homo (Quam et al. 2016), and the length along the outer surface of the cochlea is similar in Neandertals and H. sapiens (Braga et al. 2015; Beals et al. 2016). While more data are needed, there currently appears to be little change in cochlear dimensions within the genus Homo.
8.4.2 Modeling Auditory Capacities
These differences in ear anatomy have clear auditory implications, but few attempts have been made to reconstruct auditory capacities in fossil hominins (Masali et al. 1991; Moggi-Cecchi and Collard 2002). Results from these studies should be viewed cautiously since they are based on only a few ear dimensions. In contrast, a comprehensive model has been used to evaluate the auditory capacities in the early hominin taxa A. africanus and P. robustus from South Africa (Quam et al. 2015) and the Middle Pleistocene Atapuerca (SH) hominins (Martínez et al. 2004; Martínez et al. 2013). The use of models is a common practice in auditory research (Rosowski 1996; O’Connor and Puria 2008) and makes it possible to theoretically predict the flow of sound power through the outer and middle ears.
The model incorporates nearly 30 variables related to head size and the dimensions and physical properties of the anatomical structures of the outer and middle ears (Table 8.1; Fig. 8.4). In general, variables of the outer ear and ear ossicles have a stronger influence on the model results (labeled as medium and high in Table 8.1), while the middle ear spaces (tympanic cavity, mastoid antrum and air cells, and aditus ad antrum) have a much weaker influence on the results (labeled as low in Table 8.1) (Fig. 8.4). Given the important roles of both the cochlea and the brain in sound perception, the model results are not a true audiogram. Nevertheless, there is a strong correlation between sound power transmission through the outer and middle ears and auditory sensitivity to different frequencies (Rosowski 1991), and the model predicts auditory sensitivity in decibels (dB) up to 5 kHz.

Table 8.1
Modeling auditory capacities: definition of the model variables, the source of the value used, and the sensitivity analysis for frequencies above 2 kHz in the model
Electrical Parameters a | Related Anatomical Variables | ||||
---|---|---|---|---|---|
Definition | Value Used | Sensitivity b (≥2 kHz) | |||
Outer ear | Two-port network that models the concha horn | Concha length | Rosowski (1996) | High (A) | |
Cross-sectional area of wide end | Rosowski (1996) | High (A) | |||
Cross-sectional area of narrow end | Measured as AEAC | High (A) | |||
Two-port network that models the ear canal tube | Ear canal length | Measured as LEAC complete | High (A) | ||
Cross-sectional area of the ear canal | Measured as AEAC | High (A) | |||
Middle ear | Middle ear cavity | CTC | Volume of the middle ear cavity | Measured as VMEC | Low (A) |
CMC | Volume of the mastoid air spaces | Measured as VMA | Low (A) | ||
RA | Surface area of the aditus ad antrum and mastoid air spaces | Rosowski (1996) | Low (E) | ||
LA | Length and radius of the aditus ad antrum | Measured as LAD RAD1 and RAD2 | Low (E) | ||
Tympanic membrane and mallear attachment | ATM | Area of the tympanic membrane | Measured as ATM | High (A) | |
LT1 | Mass of the tympanic membrane | Estimated from ATM c | High (A) | ||
CT | Structural properties of the tympanic membrane and mallear attachment | Rosowski (1996) | Low (E) | ||
RT | Low (E) | ||||
LT | Low (E) | ||||
CT2 | Low (E) | ||||
RT2 | Medium (E) | ||||
CTS M | High (E) | ||||
RTS M | Medium (E) | ||||
Malleus, incus, ligaments, and stapes | l M :l I | Functional lengths of the malleus and incus | Measured as LM/LI | High (A) | |
LMI M | Masses of the malleus and incus | Measured as MM + MI | Medium (A) | ||
RMI M | Non-articular surface area of the malleus and incus | Rosowski (1996) | Low (E) | ||
CMI M | Structural properties of the malleus and incus | Rosowski (1996) | Low (E) | ||
LS M | Mass of the stapes | Measured as MS | Low (A) | ||
RJ M | Structural properties of the ossicular joints | Rosowski (1996) | Low (E) | ||
CJ M | Low (E) | ||||
AFP | Area of the stapes footplate | Measured as AFP | Medium (A) | ||
Inner ear | Annular ligament | CAL | Structural properties of the annular ligament | Rosowski (1996) | _ d |
RAL | High (E) | ||||
Cochlea | ZC | Structural properties of the cochlea | Aibara et al. (2001) | High (E) |

Fig. 8.4
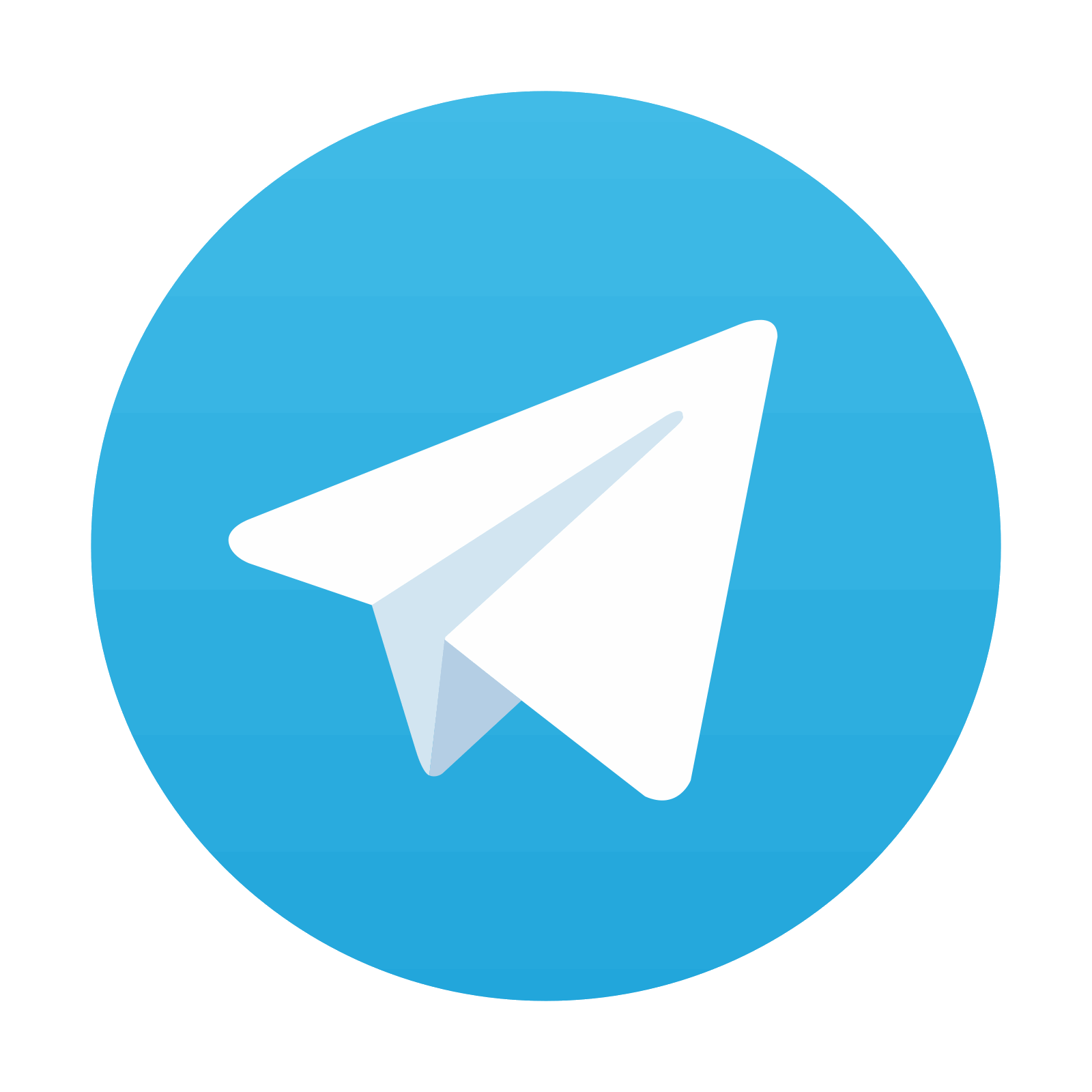
Measurements of the middle and external ears (A–C) and ear ossicles (D). A, B, C1, C2, and D are not drawn to the same scale. (A–C) are based on the 3-D reconstruction of the left side of HTB 1769 (Pan troglodytes), showing the external auditory canal (gray), the middle ear cavity (green), the aditus ad antrum (red), the mastoid antrum and connected mastoid air cells (blue), the inner ear (orange), and the temporal bone (yellow). (D) is based on the profiles of the Atapuerca (SH) ear ossicles. Abbreviations: A AD1, measured area of the exit from the aditus ad antrum (P 1 ); A AD2, measured area of the entrance to the aditus ad antrum (P 2 ); A FP , measured area of the footplate of the stapes (brown, in D); L AD , length of the aditus ad antrum; L EAC , length of the external auditory canal; L I , functional length of the incus; L M , functional length of the malleus; P 1 , plane of the exit from the aditus ad antrum; P 2 , plane of the entrance to the aditus ad antrum; P 3 , plane of the tympanic membrane; P 4 , plane marking the cross-section of the external auditory canal; R EAC1 and R EAC2, half of the measured diameters of the two major perpendicular axes of the external auditory canal; R TM1, half of the measured greater diameter of the tympanic membrane; R TM2, half of the measured lesser diameter of the tympanic membrane; V AD , volume of the aditus ad antrum; V MA , volume of the mastoid antrum and connected mastoid air cells; V MEC , volume of the middle ear cavity. (Reprinted from Quam et al. 2012; used with permission from Nova Science Publishers, Inc.)
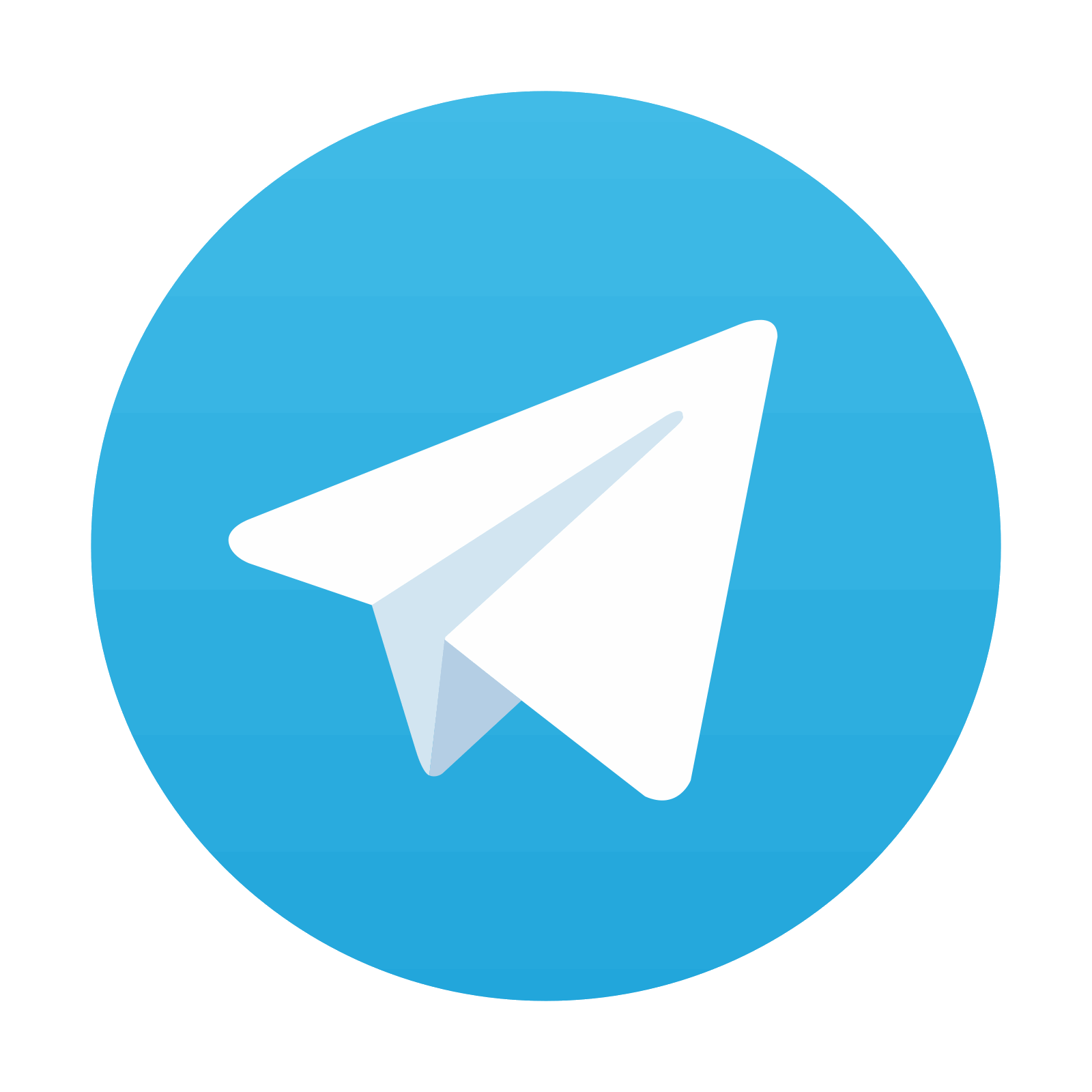
Stay updated, free articles. Join our Telegram channel
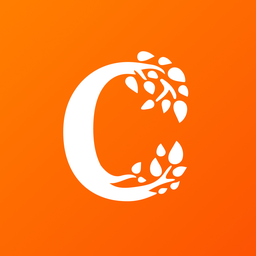
Full access? Get Clinical Tree
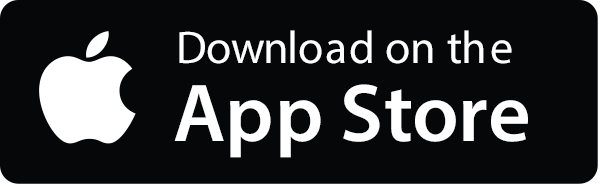
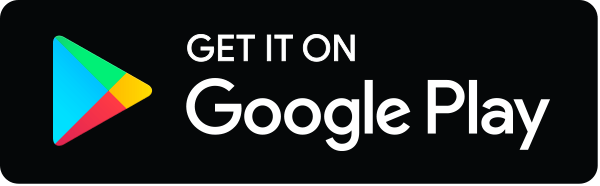
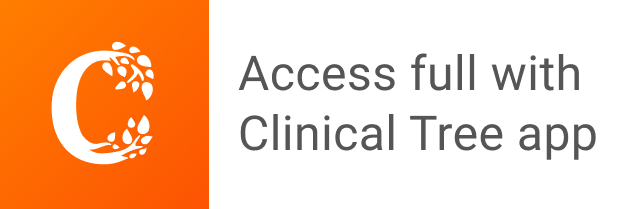