Fig. 9.1
A simplified scheme of the circadian system. The environmental photic (light) and nonphotic (feeding schedules, physical activity) zeitgebers synchronize the central circadian pacemaker (SCN) to daily light–dark and social rhythms. The SCN synchronizes other central and peripheral clocks via neuronal and humoral efferents. This internal synchronization is important for optimal physiologic function and behavior
9.2.2 Genetic Regulation of the Circadian System
Circadian rhythms are generated by a core set of clock genes, including three Period genes (Per1, Per2, Per3), Clock, Bmal1, and two plant cryptochrome gene homologues (Cry1 and Cry2) [4]. The proteins encoded by these genes interact to create a self-sustaining negative transcription–translation feedback loop that drives the timing system. The intracellular levels of CLOCK remain steady during the 24-h period. BMAL1 levels, which are high at the beginning of the day, promote the formation of BMAL1-CLOCK heterodimers. These proteins activate transcription of the PER and CRY genes resulting in high levels of these transcripts. As PER accumulates in the cytoplasm, it becomes phosphorylated and degraded by ubiquitination. Cry accumulates in the cytoplasm late in the subjective day and translocates to the nucleus to inhibit Clock-Bmal1-mediated transcription. During the night, the PER-CRY complex is degraded, and the cycle starts again. This feedback loop ensures a high level of BMAL1 and low levels of PER and CRY at the beginning of a new circadian day.
Circadian clock genes control a significant proportion of genome. It is estimated that approximately 10 % of all expressed genes are under regulation of the clock genes. Furthermore, peripheral tissues contain independent clocks. It is likely that peripheral clocks are synchronized by an input directly from the SCN or SCN-mediated messages. For a detailed overview of the molecular regulation of the circadian system, please see the excellent review by Dardente and Cermakian [2].
9.2.3 Circadian Entrainment and Markers of Circadian Rhythmicity
Circadian rhythms are synchronized with the solar day by daily adjustments in the timing of the SCN, following the exposure to stimuli that signal the time of day. These stimuli are known as “zeitgebers” (German for “time-giver”). Light is the most important and potent zeitgeber. In addition to light, feeding schedules, activity, and the hormone melatonin also influence the circadian timing [1].
Circadian rhythms can be described by their phase and amplitude. Circadian output rhythms are used to estimate the time (the phase) of the clock [5]. In humans, timing of the circadian core body temperature and hormonal rhythms are the most commonly used phase markers. The hormone melatonin is one of the best understood output rhythms of the SCN. The timing of melatonin secretion by the pineal gland is regulated by the SCN, with the onset of secretion being approximately 2 h before natural sleep time and being highest during the middle of the night [1]. Melatonin onset measured in dim light melatonin onset (DLMO) is a stable marker of circadian phase and is used in research as well as clinical practice to determine the timing of the endogenous circadian rhythm. The amplitude of a circadian rhythm refers to the half-distance from the maximum to the minimum of the observed rhythm. Plasma melatonin has been proven reliable as a marker of the amplitude of the circadian clock [6–8]. Since markers of circadian rhythms may be influenced by environmental stimuli and behavior (light–dark, rest–activity, meals), the underlying endogenous circadian rhythms are best assessed under the protocols that minimize exposure to stimuli that evoke responses in the physiological variables being monitored. The constant routine and forced desynchrony are prototypes of protocols used to assess endogenous circadian rhythms. The detailed description of these protocols is beyond the scope of this chapter.
9.3 Dopamine in the Circadian Timing System
Dopaminergic neurotransmission has been implied at several levels in the circadian system, starting with the photic afferent connection with the SCN. In the retina, dopamine (DA) plays an important role in light adaptation [9], and regulates the rhythmic expression of melanopsin, a photopigment of photosensitive retinal ganglion cells which has been implied in circadian entrainment [10]. Dopaminergic retinal cells express circadian rhythms in core clock genes Per, Cry, Clock, and Bmal1 [11]. In addition, DA modulates rhythms in retinal second messengers like cAMP, therefore interacting directly with retinal physiology and sensitivity [12]. Dopamine also affects the phase and amplitude of specific clock genes presumably through dopaminergic D1 receptors in the retina [13]. In the SCN, dopaminergic D1 receptors are present in both fetuses and adults [14, 15].
Several lines of evidence suggest that clock genes play a role in DA metabolism. The clock gene regulates dopaminergic activity in the ventral tegmental area (VTA) [16]. Several genes involved in dopaminergic signaling are differentially regulated in the VTA of Clock mutant mice, which also exhibits altered circadian rhythmicity, indicating a role of this gene in DA-related transcription in the VTA [16]. Circadian fluctuations in extracellular DA levels have been reported in the striatum and nucleus accumbens [17]. DA also regulates clock gene expression in the dorsal striatum [18, 19]. Promoter regions of the DA active transporter (DAT), D1A receptor, and tyrosine hydroxylase (TH) genes include an E-Box element, which is the target of the canonical molecular clock.
Dopaminergic activity and metabolism can also be considered an output of the circadian clock. DA and its metabolites and receptors exhibit daily fluctuations in their levels in different brain regions [20]. In particular, DA metabolism exhibits a diurnal rhythm in striatal regions, related to cyclic variations in the expression of DAT, D1A receptors, and TH [21]. There is a clear interaction between DA and melatonin, a well-known circadian output, which acts as a marker for both daily and seasonal variations in physiology and behavior, in several areas of the central nervous system [22]. Seasonal variations of DA have also been described in humans, although its importance and consequences remain to be established [23].
9.4 Circadian Rhythm Dysfunction in PD
Circadian biology of PD has not been systematically studied. Exciting emerging evidence suggests that circadian dysregulation plays an important role in PD. Answers to the question “Is PD affected by chronobiology?” require a multidirectional approach that includes a better understanding of common diurnal variations of symptoms and signs in PD as well as the assessment of physiologic and molecular markers of circadian rhythmicity in the PD population. Further, our understanding of circadian regulation in PD will be substantially enriched by examining the effects of potent modulators of the circadian system, such as light and other nonphotic zeitgebers, on PD symptoms and disease progression.
9.4.1 Diurnal Clinical Fluctuations in PD: Are they Driven by Circadian Dysregulation?
Diurnal rhythms of symptoms and signs associated with PD have been reported in numerous studies. Examples include fluctuations in daily motor activity [26–29], autonomic function [30–35], sleep–wake cycles [36–40], visual performance [41], as well as responsiveness to dopaminergic treatments [26, 42]. These observations are suggestive of possible circadian influences on the expression of clinical features of PD.
Fluctuations of motor symptoms in PD are very common. An actigraph, a wrist-worn monitor that detects movements, has been employed in numerous studies that assessed rest–activity rhythms in PD population. These actigraphy studies demonstrate lower peak activity levels and lower amplitude of the rest–activity cycle in PD patients compared to healthy older adults [36–40]. Increased levels of physical activity and shorter periods of immobility during the night result in an almost flat diurnal pattern of motor activity in PD [43, 44]. In addition, PD patients have a more fragmented pattern of activity with transitions from high to low activity periods, leading to less predictable rest–activity rhythm [45]. Motor symptoms tend to be more prominent in the afternoon and evening, both in stable patients and in those with wearing off symptoms [26, 42]. This daily pattern appears to be independent of the timing of dopaminergic medications and may be related to circadian regulation of dopaminergic systems. Furthermore, responsiveness of PD motor symptoms to dopaminergic treatments declines throughout the day, despite the absence of significant changes in levodopa pharmacokinetics [26]. While the presence of motor artifact in PD poses challenges to the interpretation of actigraphy studies in this population, actigraphy can be used in conjunction with PD sleep–wake diaries and newer nonparametric circadian rhythm analysis in order to minimize shortcoming of the actigraphy recordings of the rest–activity cycles in the PD population.
Output rhythms of the autonomic nervous system are influenced by circadian timekeeping. Alterations in the circadian regulation of the autonomic system in PD have been reported. Twenty-four hour ambulatory blood pressure monitoring in patients with PD demonstrates reversal of circadian rhythm of blood pressure, increased diurnal blood pressure variability, postprandial hypotension, and a high nocturnal blood pressure [32, 46–48]. Holter electrocardiographic monitoring in PD patients reveals a decrease of sympathetic activity during the day with a loss of the circadian heart rate variability and a disappearance of the sympathetic morning peak [31]. The spectral analysis of heart rate variability reveals a significant decrease of the daytime low frequency power, decreased daytime low–high frequency ratio, and decreased nighttime high frequency power [31, 35, 49, 50]. These abnormalities, more prominent in advanced PD, are also present in untreated patients with early PD [49]. The prognostic significance and pathophysiological mechanisms leading to altered autonomic circadian variability in PD remain to be determined. While observed abnormalities may arise within the peripheral autonomic ganglia, the influence of central networks including hypothalamus may be significant as well [51–53].
Similarly to motor performance and autonomic function, circadian fluctuations of sensory systems have been reported in PD. For example, visual performance, measured by contrast sensitivity, is altered in PD [41]. Impairment of retinal DA, which exhibits an endogenous circadian rhythm independent of light–dark cycles, is most likely responsible for these changes [54]. Since circadian changes in contrast sensitivity may occur independently of circadian oscillations in motor symptoms, it is possible that various anatomical networks (retina, striatum, and cortex) may have differential threshold to the circadian signaling of DA [55].
The sleep–wake cycle is a prototype of a biological rhythm strongly influenced by circadian system. Disruption of sleep and impaired daytime alertness are common manifestations of PD, affect majority of PD patients, and may emerge early in the course of the disease. Sleep fragmentation and excessive daytime sleepiness are the most common abnormalities of the sleep–wake cycle in PD. While the etiology of insomnia and hypersomnia associated with PD is multifactorial, circadian disruption should be considered as one of the contributing factors. Several lines of evidence stemming from research on animal models of PD support this hypothesis. Transgenic mice overexpressing human alpha-synuclein (Thy1-aSyn) exhibits disrupted circadian behaviors including the temporal distribution of sleep and activity. Furthermore, the firing rate of SCN neurons early in the progression of experimentally induced parkinsonism in the Thy1-aSyn mice may result in impairments of neural and hormonal output rhythms of the SCN.
9.4.2 Circadian Markers in PD
Physiologic and molecular markers of the circadian system may provide insight into the function of circadian timekeeping in PD. Only a few studies attempted to characterize profiles of circadian markers in PD. In a cohort of 26 PD patients, the amplitude of melatonin rhythm was decreased, and the phase was advanced in treated patients with and without motor complications compared to de novo patients [56]. These results suggest a trend toward advanced melatonin phase and amplitude reduction during the evolution of PD. Circadian rhythms of temperature and plasma cortisol did not differ between the groups in this study. In a separate study, nine patients with de novo PD had a preserved melatonin rhythm compared with healthy controls [57]. In a study of 12 PD patients, 24-h mean cortisol production rate was significantly higher and the mean secretory cortisol curve was flatter, leading to significantly reduced diurnal variation in the PD group relative to controls [58]. Body temperature is an excellent marker of endogenous circadian rhythmicity. While 24-h rhythms of core body temperature remain similar in PD relative to healthy controls [59], basal body temperature is significantly lower in parkinsonian patients [60]. PD patients with coexistent depression have altered circadian rhythms of rectal temperature and lower amplitudes of core body temperature [61]. These circadian modifications of temperature regulation have been confirmed in the 6-hydroxydopamine (6-OHDA) animal model of parkinsonism where a significant decrease of the mesor and a phase advance of temperature rhythm have been reported [62]. Although these investigations suggest modifications of circadian rhythmicity in PD, results are to be interpreted with caution due to small sample sizes and study designs that reflect influences of both endogenous circadian and exogenous (e.g., light exposure, physical activity, meals, social schedules) rhythms on PD. In a recent study of 20 patients with mild to moderate PD and 12 age-matched controls that employed modified constant routine experimental protocol that minimize exogenous influences on circadian rhythms, significant blunting of circadian rhythm of melatonin secretion and reduced amplitude of melatonin rhythm was observed [63]. PD patients with excessive daytime somnolence had a more prominent reduction in the amplitude of their melatonin rhythm compared to PD patients without EDS. Significant reductions in the amplitude of melatonin secretion was also demonstrated in de novo PD patients [64]. In contrast to these findings, Bolitho et al. recently reported a threefold increase in melatonin secretion in a cohort of PD patients [65]. While further investigations are needed, these observations provide a rationale for the role of circadian disruption in excessive sleepiness associated with PD.
Several studies have demonstrated strong oscillatory expression patterns of core clock genes in human whole blood and peripheral blood mononuclear cells (PBMCs) [66–68], demonstrating the feasibility of using clock gene expression as a circadian marker. Time-related variations in the expression of circadian clock genes have been recently reported in patients with PD [69]. In a study of 17 individuals with PD and 16 age-matched controls, the relative abundance of the clock gene Bmal1 was significantly lower during the night in PD patients versus controls. Expression levels of Bmal1 in PD patients correlated with PD severity as assessed by the UPDRS [69]. A lack of time-dependent variation in Bmal1 expression in PD patients was recently confirmed in another study [64]. Exciting emerging evidence suggests alterations in molecular regulation of circadian timekeeping in animal models of PD. Depletion of striatal DA by 6-OHDA or blockade of D2 DA receptors by raclopride blunts the circadian rhythm of striatal Per2. Furthermore, timed daily activation of D2 DA receptors restores and entrains the Per2 rhythm in DA-depleted striatum. These observations suggest a role of dopaminergic stimulation of D2 DA receptors in the maintenance of circadian molecular oscillations in the striatum.
9.4.3 Light Exposure and Nonphotic Circadian Entrainment in PD
Light, the main synchronizer for the human circadian system, is increasingly applied as therapy in a variety of sleep and neuropsychiatric conditions including circadian rhythm disorders, seasonal affective disorder, and dementia [70]. Light therapy is usually a well tolerated and easily applied nonpharmacological treatment modality. Numerous studies have demonstrated beneficial effects of light therapy on sleep quality, daytime sleepiness, mood, and quality of life in healthy elderly and in patients with dementia. Several lines of evidence support the rationale for exploring supplemental light exposure in PD. For example, DA is likely a mediator of light signaling to the retinal circadian clock which provides direct input to the SCN [9]. Exposure to light facilitates the recovery of motor function in a chronic experimental model of PD [71]. Only a few exploratory studies, however, examined the effects of bright light in patients affected by PD.
In a case series of 12 PD patients, daily exposure to supplemental bright light of 1,000–1,500 lux prior to habitual bedtime resulted in improved sleep quality and mood [72]. Evening exposure to bright light was also associated with improvements in bradykinesia and rigidity. Beneficial effects of supplemental light exposure emerged several days after initiating the treatment. Similar beneficial effects of supplemental bright light on sleep, mood anxiety, and motor performance were reported in an open-label retrospective study of 129 levodopa-treated PD patients followed up to 8 years [73]. In another study, 36 PD patients were randomized to receive treatment with bright light therapy of 7,500 lux (active arm) or 950 lux (placebo arm) in 30-min sessions every morning for 2 weeks [74]. Exposure to bright light of 7,500 lux was associated with improvements in UPDRS part I, II, IV scores, and daytime sleepiness. While these studies demonstrated good feasibility and positive effects of bright light therapy on nonmotor and motor manifestations of PD, further validation studies involving larger cohorts and employing objective outcome measures are needed. The timing, duration, intensity, and spectral qualities of light therapy will need to be optimized for use in the PD population.
9.4.4 Circadian Disruption in PD: Potential Mechanisms
Mechanisms underlying circadian dysregulation in PD are not fully elucidated. Primary neurodegenerative processes of PD leading to progressive depletion of DA likely have negative consequences on circadian timekeeping as dopaminergic transmission is an integral part of circadian homeostasis. Fluctuations in DA metabolism, overnight DA accumulation, or diurnal receptor downregulation may be in part driving diurnal fluctuations of PD clinical features [75–77]. Neuroanatomical sites of circadian disruption in PD may be along the afferent pathways to the SCN, within the SCN itself, or within the downstream peripheral efferents of the SCN. Circadian entrainment may be compromised in the population as PD patients tend to be less active and spend more time indoors. For example, reduced light exposure and/or impaired light transmission, partly due to dopaminergic retinal degeneration [78], may affect circadian timekeeping in the PD population. While the structure and function of the SCN in PD has not been rigorously examined to date, degeneration of this central circadian pacemaker may be yet another possible mechanism of impaired circadian rhythmicity in PD. Hypothalamic dopaminergic neurons, however, do not appear to be involved in the disease [79]. Finally, alterations in SCN output may be primarily responsible for fluctuations in biological rhythms and symptoms of PD.
9.5 Conclusion
Increasing evidence suggests disruptions of the circadian system in PD. Further, systematic investigations directed at circadian timekeeping may provide additional insight into the pathogenesis of daytime sleepiness and sleep dysfunction, and may possibly shed new insights into the neurodegenerative processes of PD itself. Furthermore, better understanding of the circadian biology of Parkinson’s disease may lead to the development of novel, circadian-based treatment approaches for motor and nonmotor manifestations of PD.
References
1.
Golombek DA, Rosenstein RE. Physiology of circadian entrainment. Physiol Rev. 2010;90(3):1063–102.CrossRefPubMed
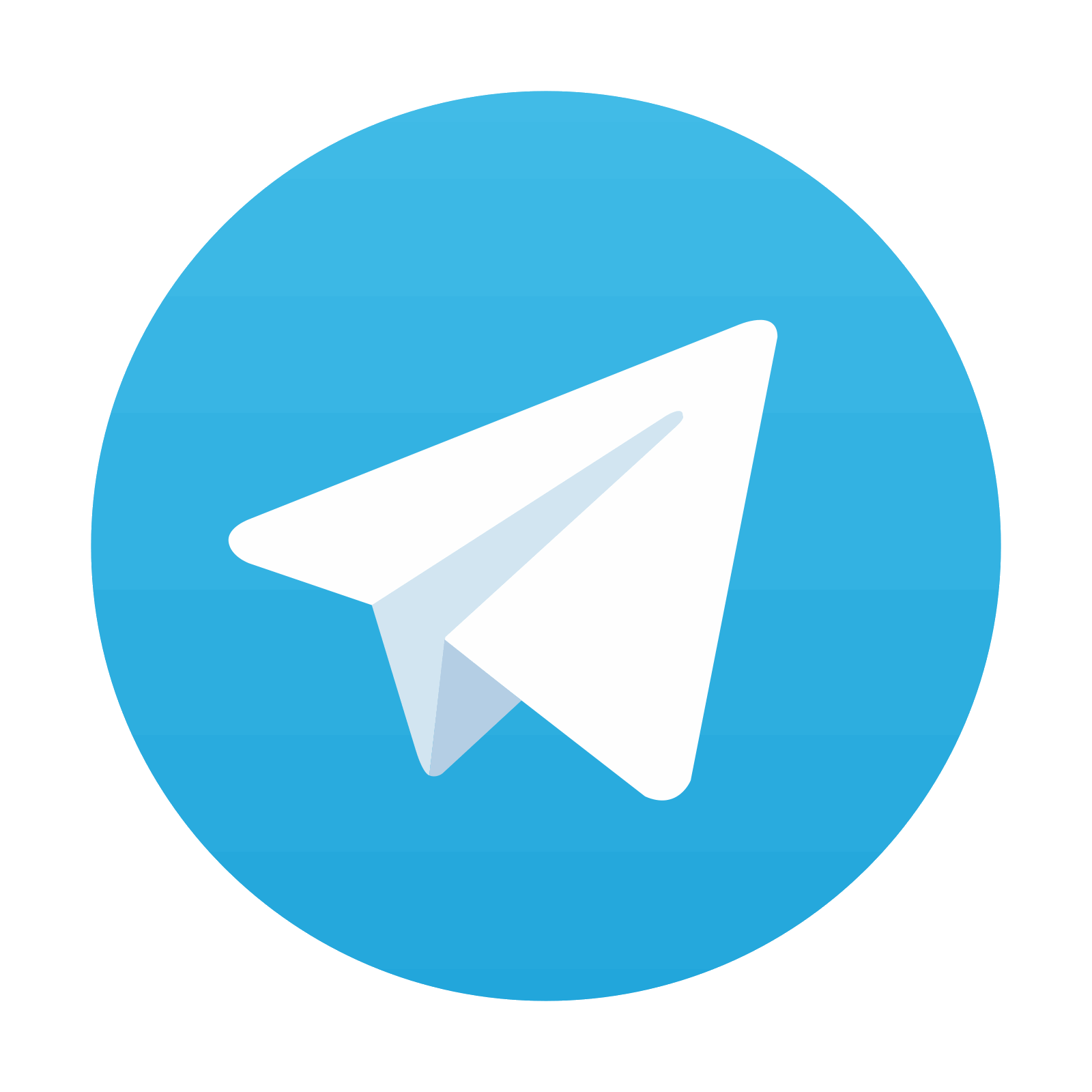
Stay updated, free articles. Join our Telegram channel
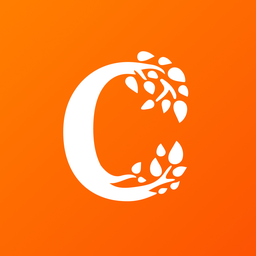
Full access? Get Clinical Tree
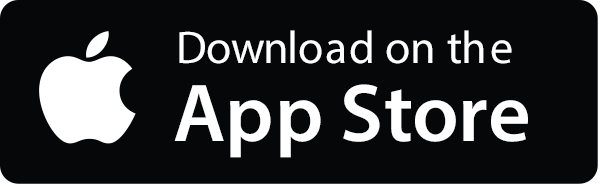
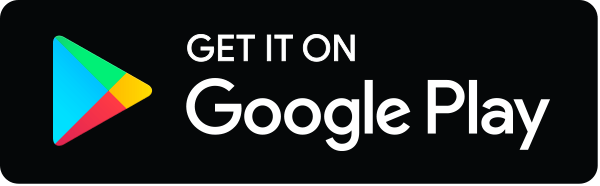