Cornea
Michael K. Smolek
Stephen D. Klyce
As a portion of the ocular tunic, the cornea protects the delicate intraocular contents with its tough, yet pliable, collagen structure. It is remarkable that a tissue with this ability to resist injury can provide the essential optics and transparency to focus an image on the retina. In this chapter the gross, microscopic, and ultramicroscopic anatomic structure of the cornea is described. Corneal physiology is not discussed, except when structural features can be more clearly defined by a description of their physiologic significance. An effort has been made to cite references primarily for normal adult human corneas; references to other species are made when human information is lacking or ambiguous. Citations to embryonic development are included when they provide better insight into the adult anatomy of the cornea.
SURFACE ANATOMY
The anterior and posterior surfaces of the human cornea are often approximated in schematic eye calculations by radii of curvature of 7.8 and 6.5 mm, respectively, compared to the external surface of the scleral globe, which has a radius of approximately 11.5 mm.1 The anterior corneal surface becomes slightly flatter in the periphery, giving the overall cornea a naturally prolate shape. If the cornea were perfectly spherical, it would suffer from considerable spherical aberration in which the rays passing through the peripheral parts of an optical system do not come to the same focal point as the rays near the central axis of the system. Peripheral corneal flattening reduces, but does not entirely eliminate, the corneal contribution of spherical aberration to the optical system of the eye. The crystalline lens provides additional correction of the residual spherical aberration of the cornea, depending on the accommodative power of the lens. The radius of curvature of the anterior surface translates into a vergence power of approximately 48.8 diopters (D), which accounts for roughly three quarters of the total refractive power of the eye’s optical system. The posterior corneal surface adds negative power so that the total power of the typical, normal cornea is approximately 43 D.
The difference in curvature between the relatively flatter anterior surface and the relatively steeper posterior surface is associated with the central cornea being thinner than the periphery. Maurice reported a central thickness value of 0.52 mm and a peripheral value of approximately 0.65 mm in adult humans when measured with an optical pachometer.2 Abnormal tissue thinning may be indicative of a corneal dystrophy, such as keratoconus, Terrien’s marginal degeneration, or pellucid marginal degeneration.
The cornea protrudes slightly beyond the limits of the scleral globe because of the difference in curvature between the relatively steep cornea and the relatively flat globe. A shallow sulcus is formed at the intersection of the corneal and scleral surfaces, which roughly demarcates a region called the limbus. The limbus is typically defined in one of two ways. The histologic limbus is the full-thickness annular interface that separates the optically transparent corneal stroma from the opaque sclera. The surgical limbus is the annular region bound by a line from the anterior surface termination of Bowman’s layer to the posterior surface termination of Descemet’s membrane and by a line oriented perpendicular to the external scleral surface that intersects Schlemm’s canal in the angle of the anterior chamber.
Externally, the cornea appears elliptical with its vertical chord shorter than its horizontal chord (10.6 versus 11.7 mm for males and 9.6 versus 10.7 mm for females).2 This difference arises from opaque scleral tissue extending over the anterior corneal margin slightly more along its superior and inferior aspects. When viewed from within the dissected globe, the posterior cornea appears circular with a diameter of 11.7 mm. The external corneal surface area is approximately 120 mm2, or one-fourteenth the total area of the ocular globe.3 Surprisingly, the surface area is not significantly increased by the localized distension found in keratoconus, although keratoglobus conditions do appear to have a greater surface area.
TEAR FILM
The precorneal tear film is approximately 7 μm thick with a volume of 6.2 ± 2 μL during normal tear production.4 Tear fluid is typically produced at a rate of 1.2 μL/minute, with a major portion drained from the palpebral fissure through the nasolacrimal duct and a smaller volume lost through evaporation from the ocular surface (approximately 3 μL/hour at 30% relative humidity).5 Tear chemistry is complex; ingredients include various electrolytes, metabolites, proteins, enzymes, and lipids.6 The functional significance of the tear film is broad. It provides lubricating qualities and a smooth optical interface with the air. It also protects the epithelium from airborne contaminants and provides natural immunity to infectious agents through secretory immunoglobulin molecules.7
The tear film is composed of the cellular exudates of separate structures of the ocular adnexa and has been difficult to measure precisely because of its delicate fluid nature.8 The anterior-most layer is the lipid or oily layer derived from secretions of the meibomian glands located in the eyelid and caruncle and is between 0.1 and 0.5 μm thick. The aqueous lacrimal tear layer is at least 5 μm thick and often thicker, depending on tear production by the lacrimal glands located in the superiotemporal margin of the orbit. The posterior mucous layer is approximately 1 μm thick and is derived from secretions of conjunctival goblet cells. The hydrophilic nature of mucus substantially reduces surface tension and provides a smooth, wettable surface for the aqueous tear layer. The separation between the middle lacrimal tear layer and the posterior mucous layer is actually indistinct, making definitive thickness measurements of the two components difficult. It is highly likely that these two components form a graded mixture, with the posterior mucous component gradually blending into the anterior aqueous lacrimal tear component.
EPITHELIUM
The corneal epithelium is the anterior-most cell layer of the cornea (Fig. 1). It is typically several cell layers thick, consisting of the apical cell squamous layer, the multilayered, polygonal-shaped wing cells beneath the apical layer, and the posterior-most layer of basal cells (Fig. 2). The wing cell layer is two or three cells thick in the central cornea, but tends to be four to five cells thick in the periphery. In total, the epithelium is approximately 50 μm thick in the central human cornea.
The epithelium is in a constant state of turnover, with exfoliating apical cells being replaced by underlying wing cells. During normal exfoliation, desquamating cells are released only after the replacement cell has established new tight junctions with neighboring cells and the new apical membrane is capable of maintaining continuity of the tear film.9 Studies of induced exfoliation of a monolayer of epithelial cells with a biologic detergent indicate recovery of the paracellular barriers and transepithelial electric resistance in approximately 1 hour.10 The epithelium completely turns over in approximately 7 days.11 Once injured, a high degree of motility ensures coverage of a denuded area by adjacent basal cells, followed by replacement of the normal complement of cell layers. Basal cells are the only epithelial cells capable of mitosis; however, many epithelial cells originate as the progeny of limbal stem cells and migrate centripetally to supplement or replace cells lost through normal desquamation or injury.12,13,14 Using immunohistochemical staining for antibodies to keratins, Wiley and associates found regional heterogeneity indicating that the superior corneal periphery and limbus have the greatest numbers of stem cells producing replacement epithelial cells.15 Limbal stem cell deficiency may result in conjunctival epithelium invasion of the cornea, leading to vascularization, the appearance of goblet cells, and an irregular or unstable epithelium that reduces visual acuity and may produce pain or discomfort.16
The epithelium is known to chemically interact with keratocyte cells of the stroma. These interactions appear to be dominated by cytokines such as interleukin-1 (IL-1) and soluble Fas ligand that are released by injured epithelial cells. It would appear that IL-1 is a master regulator for corneal wound healing given its effect on keratocyte apoptosis and the modulation of matrix metalloproteinase and growth factors such as keratinocyte growth factor (KGF) and hepatocyte growth factor (HGF). The Fas ligand system is known to influence the immune privileged state of the cornea. In addition to the epithelial-to-keratocyte communication, keratocytes influence the state of the epithelium via HGF and KGF, which affect cell turnover, motility, and proliferation.17
APICAL CELLS
Apical surface cells appear broad and flattened: 4 to 5 μm thick and 40 to 50 μm in diameter. Freshly emerged surface cells appear bright during specular microscopy and have relatively small numbers of microvilli covering their apical membrane (Fig. 3).18
![]() FIG. 3. Scanning electron micrograph of corneal epithelium surface. Note the numerous microvilli and the cellular margins (12,900×).(Courtesy of Drs. Rodrigues, Waring, Hackett, and Donohoo.) |
As the cell matures, its specular microscopic appearance tends to darken (Fig. 4). This may be due to changes in surface texture, because microvilli densely cover the apical membrane at this stage of development.4,19 Prior to cell exfoliation, apical surface margins tend to appear smooth, with microplicae clustered only near the center. In its final stages the biomicroscopic appearance of the cell surface appears darker than in its earlier stages (Fig. 5).
![]() FIG. 4. Confocal microscopic transverse image of the human surface epithelium in vivo. The squamous cell nuclei and some cell margins are visible (500×). (Courtesy of Nidek Technologies.) |
The 300-nm thick glycocalyx (buffy cell coat) of the apical membrane can be preserved intact for histologic evaluation.7,8,19 The glycocalyx is composed of glycoprotein material, and numerous separate fine filaments become visible on the apical surface after tannic acid staining (Fig. 6). These filaments cover the tips and sides of the microplicae and microvilli extensively, inserting into the cell membrane. Angular bends and filament branching are evident, as well as a beaded substructure.19 The shortest filaments are 150 nm in length in the central cornea, while filaments 300 nm long are found on the conjunctival cell surfaces.19 The glycocalyx binds loosely with the overlying mucous layer and provides binding sites for immunoglobulin present in the tears.7
The margins of the apical cell membrane possess the important tight junctions surrounding the cell circumference near the apical margin.20 This junction complex is the correlate of the paracellular pathway of high resistance to ion flow. The lateral and basal membranes of the apical cells have gap junctions, numerous desmosomal junctions, and numerous membrane-bound vesicles. The cytoplasm contains a flattened nucleus (which probably disintegrates prior to desquamation), few organelles, and a notable increase in tonofilaments compared with the underlying cells. There are some aggregates of glycogen granules, small mitochondria, sparsely distributed free ribosomes, and poorly developed Golgi’s complexes. Cytoplasmic vesicles are fairly numerous.21
WING CELLS
Wing cells are distinguished by a variety of polygonal shapes and by their large ovoid nuclei. The cells are roughly 12 to 15 μm in diameter, and their cytoplasm contains few rough endoplasmic reticulum cisternae, mitochondria, or Golgi’s complexes. The large numbers of cytoskeletal tonofilaments are approximately 8 nm in length, and numerous interdigitations exist along the cell membranes.21 Desmosomal and gap junctions are seen between adjacent wing cells and between basal and apical cells (Fig. 7).
BASAL CELLS
Basal cells appear as elongated polygonal cells approximately 10 μm in width and 15 to 20 μm in height, with prominent ovoid nuclei. The polygonal nature can be readily discerned in confocal microscopy images of the basal cell layer (Fig. 8). Buschke and associates22 showed that cell mitosis occurs in only 1 of 250 basal cells of the rat epithelium. In their study, mitosis occurred in irregular clumps of three to six cells, and mitotic cells were much more numerous in the periphery. The basal cell cytoplasm appears similar to that of the wing cells, as do the anterior and lateral cell membranes with their complement of desmosomal attachments. However, the basal membrane is notable for the presence of hemidesmosomes, which are discussed below.
ANCHORING STRUCTURES
HEMIDESMOSOMES
The epithelial basal cell hemidesmosome is a multilayered junctional structure associated with the basal membrane, with intracellular keratin filaments (intermediate filaments) entering along its cytoplasmic aspect. As basal epithelial cells migrate in response to wound healing, hemidesmosome junctions are observed to disassemble and their integrin components diffusely distribute across the basal cell membrane.23 In vitamin A-deficient rat models, the severity of epithelial sloughing was found to correspond to the size and frequency of residual hemidesmosomes.24
Kurpakus and colleagues25 found a monoclonal antibody, mAb6A5, that was directed against a 200-kd polypeptide component on the cytoplasmic exposure of the hemidesmosome. This component may be associated with the electron-dense plate that is seen crossing through the intermediate filaments just proximal to the hemidesmosome plaque.26 Short filaments pass from the dense plate into the plaque. Polypeptides of 180 and 230 kd were isolated by Owaribe and co-workers26 and localized to the hemidesmosome plaque. The 230-kd component was found to be the bullous pemphigoid antigen (BPA), while the 180-kd component was heavily glycosylated, making it a candidate for a transmembrane glycoprotein. In addition, major polypeptides of 120, 200, and 480 kd were isolated from the region and may be hemidesmosome structural components as well.26
Stepp and associates,27 using direct immunofluorescence of frozen sections, found β1, β4, and β5 integrin subunits and α3, α5, α6, and αv subunits localized in the epithelium. Specifically, α5, α6, and β4 subunits of integrin heterodimers were found to be localized on the basal membrane side of the hemidesmosome site, while β1, β5, α2, α3, and αv were localized to sites associated with cell-t-cell contact.27 A 125-kd polypeptide was isolated to the basal side of the hemidesmosome by Kurpakus and co-workers28 with the monoclonal antibody mAbHD. This polypeptide may be a protein associated with the anchoring filaments that span the lamina lucida from the hemidesmosome plaque to the basal lamina.
Fluorescence confocal imaging of the integrin subunits α6 and β4 indicated that the α6 subunits were localized to both the basal and lateral surfaces of the basal epithelial cell, but the β4 subunits were present only along the basal floor of the cell. The number and distribution of hemidesmosomes do not appear to vary with age; however, discontinuous staining for the α6 subunits in corneal epithelium of individuals over the age of 70 has been noted.29
BASAL LAMINA
Basement membrane is secreted extracellularly by the epithelial cells and forms one of several structural components associated with cell adhesion to Bowman’s layer or stroma. With light microscopy, a densely stained basement membrane approximately 75 to 100 nm thick is visible.21 Under the high magnifications of electron microscopy, this layer appears distinctly laminated. Anteriorly, the lamina lucida appears as a clear, electron-lucent zone approximately 23 nm thick, while the lamina densa that lies apposed to Bowman’s layer is an electron-dense region approximately 48 nm thick.21 It has not been established how basal lamina adheres to Bowman’s layer. A third component of the basement membrane is the reticular lamina, which lies distal to the lamina densa and is within Bowman’s layer. This region includes the anchoring fibrils and plaques and other electron dense materials associated with the lamina densa (Fig. 9).
Numerous electron-dense, fine filaments are observed clustered within the lamina lucida; they course from the hemidesmosomes of the basal epithelium and insert into neighboring regions on the lamina densa. Within the lamina lucida, a dense plate often can be seen interposed along the filaments and lying parallel to the hemidesmosome plaque. The precise composition of these filaments and the dense plate is uncertain, but the 125-kd polypeptide described previously may be involved.28 In addition, the antihuman monoclonal antibody, 19-DEJ-l, was found to be reactive to the antigenic epitope of either the anchoring filaments or the dense plate through the filaments.30
ANCHORING FIBRILS AND PLAQUES
Within the anterior aspect of Bowman’s layer, fine filaments travel distally from the lamina densa near the proximal insertion of the anchoring filaments and immediately coalesce to form striated anchoring fibrils approximately 0.15 μm in width (Fig. 10).31 These anchoring fibrils then course posteriorly to an average depth of 0.60 μm in the adult human and terminate in electron-dense regions called anchoring plaques.31,32 Maximum penetration depths of 2.05 μm were reported for the anchoring fibrils in humans. Some anchoring fibrils may terminate among the type I collagen fibrils31 or return proximally to reinsert into the lamina densa at a neighboring site.32 There also appear to be plaque-to-plaque anchoring fibrils. Anchoring fibrils found in Bowman’s layer appear similar to those found in the stroma of the rabbit, a species that lacks Bowman’s layer.31
Anchoring fibrils were found to be type VII collagen filaments in bovine32 and human corneas31 using immunogold labeling and immunofluorescence staining techniques, respectively. Type VII collagen is a dimer of high molecular weight.33 Each monomer has a helical domain and a globular, carboxyl domain. The dimer is created by disulfide bonds linking the tails of the monomers together, and striated fibrils are formed by the nonstaggered arrangement of the helical filaments. The globular domains were found localized within the anchoring plaques, which also label for type IV collagen.30 Gipson and co-workers31 and Keene and colleagues32 presented similar models for the architecture of the anchoring fibril network and indicated that the striated type I collagen fibrils in Bowman’s layer must be interwoven and trapped by the anchoring fibril network (Fig. 11).
BASEMENT MEMBRANE CONTROVERSY
Basement membranes throughout the body are formed primarily from type IV collagen, yet evidence for type IV collagen within corneal lamina densa remains sketchy and controversial. Marshall and associates were unable to demonstrate the presence of type IV collagen in the human basal lamina using immunoelectronmicroscopy labeling with colloidal gold.34 Indirect immunofluorescence labeling for anti-type IV antigens was shown within a subepithelial band by Konomi and colleagues,35 but the layer did not appear strongly labeled. Newsome and co-workers36 indicated strong immunofluorescence labeling, but the corneal location was not specified. Nakayasu and associates37 showed strong immunofluorescence labeling of a subepithelial layer near the limbus but did not report type IV labeling for the concomitant immunoelectronmicroscopy portion of the study.
In a project designed to resolve this controversy, Kolega and colleagues38 found that basement membrane type IV collagen was differentially stained according to location in the adult human. There was no immunofluorescent staining for type IV collagen in the central cornea using three different monoclonal antibodies, and only weak staining in the corneal periphery. However, there was intense staining in the conjunctiva and across the limbal region. Cleutjens and colleagues39 and others40,41 also found no type IV collagen in the central cornea of the adult but did find it in the periphery. Furthermore, type IV collagen was found to be strongly labeled in the fetal central cornea.39
Linsenmayer and associates42 had previously discussed findings of heterogeneous type IV collagen distribution in a variety of basement membranes throughout the body, including the crystalline lens capsule.42 Thus, it would be unusual, but not unexpected, for corneal epithelial basement membrane to exhibit a heterogeneous distribution. If type IV collagen is missing, binding mechanisms in the lamina densa would have to be reconsidered. This also would have implications for how laminin, heparan sulfate proteoglycan, and fibronectin binding operate in the absence of type IV collagen.
It is possible that the type IV collagen present in the human cornea does not react to any anti-type IV antibodies known to date.43 A second possibility may be that type IV collagen is masked to specific immunolabels by proteoglycans surrounding the collagen.40,41,44 Finally, type IV collagen that is seen in the embryologic basement membrane may diminish with maturation of the tissue. Cleutjens and co-workers39 have stated that the underlying Bowman’s layer, because it is acellular, may not provide the appropriate signals to the epithelium to stimulate type IV production. Any type IV that is present could be either remnant embryonic material or produced by progeny cells of the limbal stem cells. This would explain the differential localization of type IV and indicate that progeny cells of the stem cells lose their ability to produce type IV collagen during migration toward the central cornea.
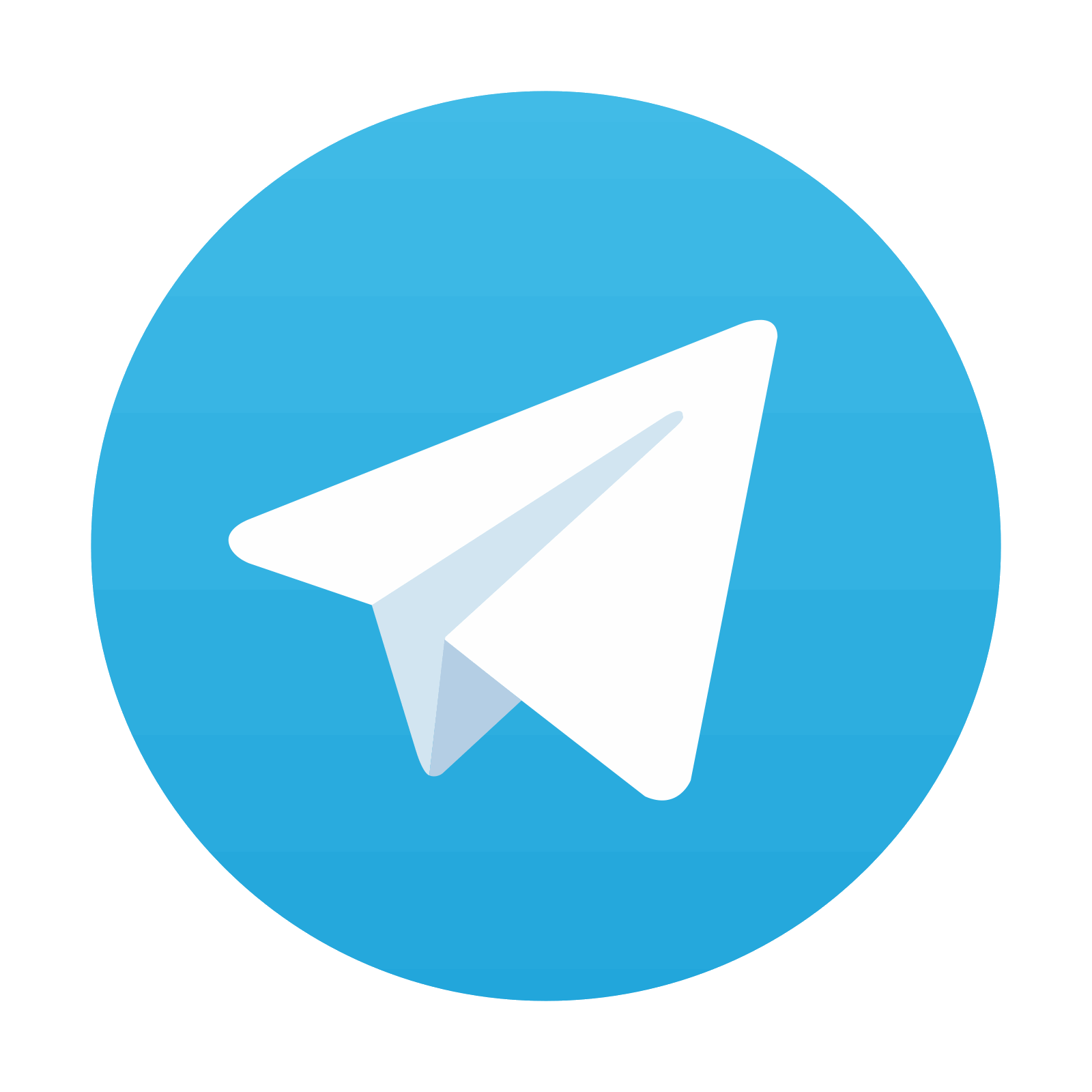
Stay updated, free articles. Join our Telegram channel
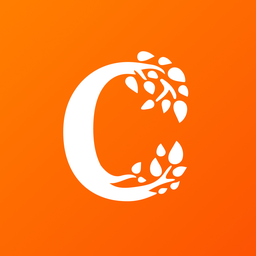
Full access? Get Clinical Tree
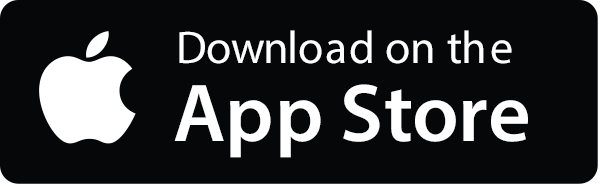
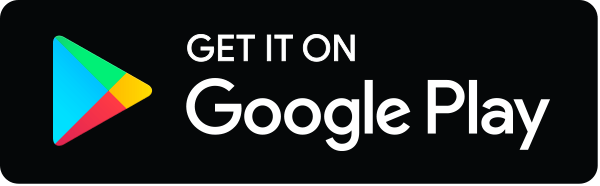