3
Consequences of Deafness and Electrical Stimulation on the Peripheral and Central Auditory System
Robert K. Shepherd, Noah E. Meltzer, James B. Fallon, and David K. Ryugo
Cochlear implants provide important auditory cues necessary for auditory awareness and speech perception in severe to profoundly deaf subjects. Over the past three decades more than 80,000 adults and children worldwide have received these devices. Clinical experience has shown, however, a large variability in outcome among implant users. Factors predicting a successful clinical outcome reflect the importance of auditory experience—either before an acquired hearing loss or with use of a cochlear implant. Moreover, deaf children, with little or no prior auditory experience, can obtain significant benefit from a cochlear implant provided that their device is fitted at a young age. This clinical experience suggests that such a response can be at least partially attributed to plasticity within the auditory system.
This chapter reviews the response of the auditory system to both deafness and its reactivation through a cochlear implant, and includes experimental data from animal models as well as human material where applicable. Understanding the complexities of this response will help provide a substrate for understanding the clinical variability evident among cochlear implant users.
♦ The Cochlea
Cochlear Pathophysiology Following Deafness
Spiral ganglion neurons (SGNs), whose cell bodies are located within Rosenthal’s canal (Fig. 3–1), are the target neurons for cochlear implants. These devices electrically depolarize local populations of SGNs, initiating the generation of action potentials via an electrode array typically located in the scala tympani (a description of the initiation of an action potential via an electrical stimulus is provided elsewhere1). It is important to emphasize that once the action potential has been initiated, the implant plays no further role in its propagation along the ascending auditory pathway; saltatory conduction along the central process of the SGN and its passage across synapses within the cochlear nucleus (CN) or higher auditory centers are performed via normal physiologic processes.
Survival of these neurons is dependent on the integrity of the organ of Corti; SGNs are subject to atrophic and degenerative changes that occur secondary to a sensorineural hearing loss (SNHL). Although the minimum number of SGNs required to achieve acceptable clinical performance with a cochlear implant is unclear, the greater number of viable SGNs available for stimulation is likely to result in improved clinical performance.2,3 Here we discuss the pathophysiologic changes that occur to the cochlea following SNHL and describe techniques that may lead to SGN preservation.
Cochlear Pathology
Hair cells of the organ of Corti are sensitive to many forms of pathologic damage including acoustic trauma, ototoxic drugs, congenital abnormalities, and aging. Unlike avian hair cells, mammalian hair cells cannot spontaneously regenerate; loss of hair cells results in a permanent SNHL. Several atrophic and pathologic changes occur to SGNs following the loss of the sensory epithelium and the support cells of the organ of Corti. First, there is a rapid and extensive loss of the unmyelinated peripheral processes within the organ of Corti.4 This process is followed by a more gradual degeneration of the myelinated portion of the peripheral processes within the osseous spiral lamina and of the cell bodies within Rosenthal’s canal5–7 (Fig. 3–1). The degenerative process also results in demyelination of residual SGN soma and possibly part of their central processes.5,7,8 Finally, the perikaryon of residual SGNs undergoes considerable shrinkage,9–11 a response that is repeated throughout the central auditory pathway following an SNHL (see below), although the functional effects of this atrophy are unknown.
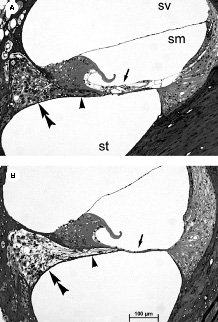
Figure 3–1 Photomicrographs of the upper basal turn from (A) normal hearing and (B) 10-week deafened rat cochleae. The normal organ of Corti evident in the control animal contrasts with the complete degeneration of both hair cells and their supporting structure in the deafened cochlea (arrow). Other deafness-induced degenerative changes evident here include a loss of peripheral processes that would normally innervate the organ of Corti (arrowhead), and loss of the spiral ganglion neuron (SGN) soma within Rosenthal’s canal (double arrowhead). sv, scala vestibuli; sm, scala media; st, scala tympani.
The secondary degeneration of SGNs following loss of the organ of Corti is an ongoing process, eventually resulting in very small numbers of surviving neurons6,7 (Fig. 3–2). As we will describe below, these pathologic changes affect the physiologic response of SGNs to an electrical stimulus. The ongoing degeneration of SGNs has been widely reported across mammalian species following various etiologies that target the organ of Corti: mouse,12 rat,13,14 chinchilla,15 guinea pig,16 and cat.6,7,17–21 It is important to emphasize that the rate of SGN degeneration exhibits considerable variation across species—a theme we shall return to when discussing SGN loss in humans.
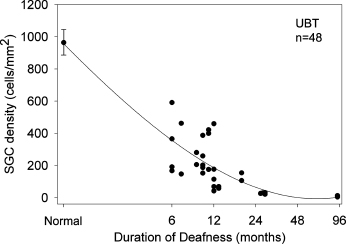
Figure 3–2 Graph illustrating the rate of loss of SGNs in the upper basal turn (UBT) of the cat cochlea as a function of duration of deafness. Although there is a relatively wide individual variability in the data, there is a clear reduction in SGN survival with increased durations of deafness. Similar degenerative patterns are observed in other mammals albeit at different rates. Although rodents such as guinea pigs exhibit a more rapid loss of SGNs compared with the cat, it is important to emphasize that ganglion cell loss is significantly slower in humans. These data are based on counts from 48 cat cochleae including 16 normal hearing controls. Error bar = +/−1 SEM.
In the majority of forms of SNHL, SGN degeneration is secondary to the loss of hair cells and support cells of the organ of Corti.22–24 These changes appear due, at least in part, to the withdrawal of neurotrophic factors normally expressed by the hair cells25–38 and nerve growth factors released by support cells.29 This pathway of SGN loss is common to many forms of SNHL described clinically, and would be expected to result in a gradual but ongoing secondary degeneration of SGNs as reported above. Importantly, etiologies that directly target SGNs, including viral and bacterial labyrinthitis, mechanical trauma, and disruption to cochlear vasculature, are likely to result in a more rapid and extensive loss of auditory neurons.
Reports of degeneration patterns of SGNs in human cochleae following a profound SNHL are generally consistent with the experimental studies already summarized. Degeneration of peripheral processes is more extensive than that of the SGN soma or central processes, and the extent of the pathology typically varies with distance along the cochlear partition in a manner that reflects the extent of damage to the organ of Corti, being most extensive in the basal turn with less extensive pathology apicalward.3,22,23,30–33
Analysis of SGN survival from profoundly deaf adult temporal bones reveals a moderate to severe loss of auditory neurons. Otte and colleagues32 studied 62 profoundly deaf ears and demonstrated that 45% of cochleae had a SGN population in excess of 10,000, that is, approximately one third of normal (28,418 ± 3,6753). Nadol and colleagues3 studied 66 profoundly deaf ears and showed that the mean SGN population was approximately half that of normal ears, although the standard deviation, as in all studies of this sort, was large (14,061 ± 8,063). Total SGN loss tended to be greater in older compared with younger subjects and, consistent with animal studies, greater loss was observed with longer durations of deafness. However, the single most significant determinant of SGN loss in humans was etiology,3,31 with loss most extensive in patients with postnatal viral labyrinthitis, congenital or genetic deafness, or bacterial meningitis, that is, pathologies that directly target SGNs or patients having long durations of deafness. Patients deafened with aminoglycoside antibiotics or sudden idiopathic deafness typically exhibited the smallest levels of SGN pathology.
Although many of the findings observed in adult temporal bones have also been reported in temporal bones from profoundly deaf children, two important differences were noted: (1) the SGN population in children did not show evidence of ongoing loss with duration of deafness within the age range of 0 to 9 years; and (2) there was a more even distribution of SGNs throughout the cochleae.34 These results provide encouraging findings for pediatric cochlear implantation, and emphasize the relatively slow rate of SGN degeneration evident in humans compared with experimental animals. This situation may reflect, at least in part, the lack of a long-term decrease in clinical performance with device use.35
Physiologic Response of the Spiral Ganglion Neuron to Deafness
Despite the extensive pathologic changes that occur to SGNs following an SNHL, these neurons remain capable of initiating and propagating action potentials elicited via an electrical stimulus, even in cochleae deafened for many years, with surviving neural populations of <5% of normal.6 In general, the basic response properties of SGNs in deafened cochleae remain similar to those observed in normal cochleae; that is, the cells show an increase in the probability of firing and a decrease in both response latency and the jitter in the timing of the response with increasing stimulus current. There are, however, more subtle changes seen in neural response properties in cochleae subjected to long periods of deafness; these pathology-induced changes have the potential to degrade the perceptual quality of cochlear implants. First, loss of peripheral process and ongoing loss of SGNs results in an increase in threshold.14,36–38 This change likely has an adverse effect on power consumption and results in a reduction in spatial selectivity of the electrode array.39 Second, demyelination results in an increase in membrane capacitance,40,41 reducing the efficiency of a neuron in initiating and propagating action potentials in response to electrical stimuli. In the auditory system, there is evidence of reduced temporal resolution in myelin-deficient or deafened cochleae,42 as well as a significant increase in the refractory properties of auditory nerve fibers14 and evidence of conduction block.17
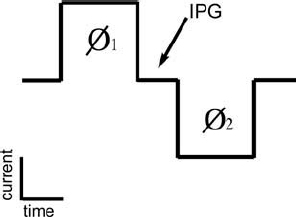
Figure 3–3 Schematic diagram illustrating the charge balanced biphasic current pulses typically used in cochlear implants. Charge in the second phase (Ø2) is equal in magnitude but opposite in polarity to the charge in the first phase (Ø1). The duration of each phase typically varies between ˜10 and 50 μs/phase for monopolar stimulation and 50 and 200 μs/phase for the less efficient bipolar stimulation. IPG, interphase gap.
Effects of Electrical Stimulation
Safety Issues
Nondamaging electrical stimulation consists of short-duration (<200 μs/phase) charge-balanced biphasic current pulses (Fig. 3–3) delivered using platinum electrodes and operating at charge densities of <60 μC/cm−2 geom per phase.43–49 These stimulus guidelines ensure that the charge injection process is achieved using reversible electrochemical reactions localized to the electrode–tissue interface, minimizing the chance of releasing potentially harmful electrochemical products into the tissue environment.50–52 Contemporary cochlear implants operating in a monopolar electrode configuration would typically produce charge densities of an order of magnitude below these levels.
Although theoretically, charge-balanced biphasic current pulses should not result in the production of potentially damaging direct current, in practice it is not possible to generate perfectly balanced stimuli. Neural damage and new bone formation is observed following chronic stimulation with direct current levels greater than 0.4 μA.53,54 Protection against direct current, and the local pH changes that occur as a result of charge imbalance, can be achieved by either shorting electrodes between current pulses or by placing a capacitor in series with each electrode.55,56 One or a combination of these techniques is used to ensure complete charge recovery in contemporary cochlear implant systems.
Trophic Effects of Electrical Stimulation on Spiral Ganglion Neuron Survival
In deafness, there is a lack of both driven neural activity and significant reductions in the levels of spontaneous activity,17,20,21,37 such that SGNs rarely undergo depolarization. However, neural activity is known to play an important role in SGN survival; depolarization is sufficient to maintain neuronal survival in vitro without the addition of neurotrophic factors.57 Membrane depolarization appears to promote SGN survival by elevating intracellular calcium levels and cascading several intracellular signaling pathways.57 Finally, the trophic support of SGNs via depolarization in vitro appears to be additive with the actions of some neurotrophins.58
Several in vivo studies have described significant increases in SGN survival—to levels of up to 70% greater than non-stimulated control cochleae—in ototoxically deafened animals following chronic electrical stimulation of the auditory nerve.59–67 However, it is important to emphasize that this is not a universal finding; work from other laboratories has reported no such trophic influence.43,45,68,69 Given the subtle methodological differences across studies, this variation in results is not surprising. Indeed, it is expected that these differences will ultimately provide further insight into the mechanisms underlying stimulus-induced trophic support of SGNs.
There is agreement, however, that chronic electrical stimulation in deafened ears results in subtle morphologic changes to SGNs. For example, there is a small but significant increase in the soma area of SGNs in deafened, chronically stimulated cochleae compared with deafened controls.43,67 This increase in soma area presumably reflects an increase in biosynthetic activity within the SGN soma following reactivation via an electrical stimulus.
Neurotrophin Support of the Spiral Ganglion
Several growth factor families have been shown to play important roles in the development, maintenance, and protection against injury of SGNs.70 Both presynaptic hair cells and postsynaptic neurons within the CN are necessary for SGN survival, reflecting complementary neurotrophic support from both sources. These neurotrophins include both brain-derived neurotrophic factor (BDNF) and neurotrophin-3 (NT-3)25,26,71 , with receptors for both of these neurotrophins expressed on SGNs.25,26 Moreover, survival of SGNs in the absence of hair cells has been promoted by exogenous BDNF or NT-3 in vivo.13,16,72–74
This research has recently been extended to demonstrate enhanced SGN survival in deafened cochleae treated with both exogenous neurotrophins and electrical stimulation63,69 (Fig. 3–4). Importantly, this work has also shown a functional advantage, in the form of significantly reduced electrically evoked auditory brainstem response thresholds in ears treated with neurotrophins,69,75 that may be associated with a neurotrophin-mediated growth of SGN peripheral processes toward the scala tympani.74,76 Reductions in electrical thresholds would reduce the power consumption of a cochlear implant, thereby providing several engineering advantages including the possibility of smaller, more numerous electrode contacts.
Although these results are promising, from a clinical perspective further research is necessary before neurotrophins can be combined with cochlear implants. First, the long-term safety and efficacy of neurotrophin delivery to the cochlea must be examined, as there is evidence that the removal of exogenous BDNF leads to an accelerated loss of SGNs,77 implying that the neurotrophin must be supplied continuously. The perilymph of scala tympani is connected to the cerebrospinal fluid via the cochlear aqueduct; therefore, safety studies must be directed at both the level of the cochlea and the central nervous system (CNS). Second, an appropriate strategy for long-term delivery of neurotrophins must be established. The use of pumps should be discouraged because of the risks of introducing infection into the inner ear. Alternative delivery methods using viral vectors or cell-based therapies hold promise in this area.63 Finally, while neurotrophin therapy has been shown to protect SGNs following an insult to the organ of Corti, it is not clear whether neurotrophins would be effective in etiologies that directly target SGNs.
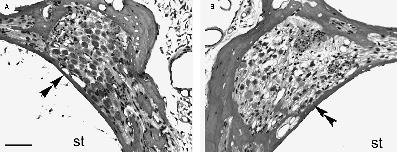
Figure 3–4 Photomicrographs of Rosenthal’s canal (double arrow-head) and the SGNs of the upper basal turn from deafened guinea pig cochleae (A) treated with the neurotrophin brain-derived neurotrophic factor (BDNF) and electrically stimulated via a cochlear implant and (B) the contralateral deafened untreated control. The near-normal SGN population in the BDNF-treated cochlea contrasts with the ˜50% SGN loss after 4 weeks of deafening in the deafened control cochlea. st, scala tympani. Scale bar = 50 μm.
♦ The Cochlear Nucleus
Deafness and the Cochlear Nucleus
The role of acoustically generated activity on the structural and functional development of the central auditory system is a subject of intense research. Auditory deprivation in the developing animal produces severe abnormalities in the central pathway, whereas older animals are less affected.78–84 Postmortem studies of those rare human cases where the profound hearing loss of the individual is documented has revealed up to 50% somatic shrinkage in the CN, with acknowledgment that many variables contribute to the nonuniform results.85–88 Clinical observations related to congenital deafness suggest that the best candidates for cochlear implants are very young children, and that with increasing age the outcomes become less optimal.89–91 The implication is that sensory stimulation, whether natural or prosthetic, is necessary during early life to ensure the normal development of the central auditory system.
In the auditory system, there is a period during which the normal development of speech and hearing is adversely affected by sound deprivation, and thereafter it cannot be remedied even if sound is fully restored. The nature of this epoch, called the “critical period,” is conceptually similar across species but undoubtedly varies in timing and magnitude of effect. Intervention strategies in the case of pediatric patients with a profound SNHL have concentrated on cochlear implants, and there is a growing tendency to implant deaf children at earlier and earlier ages. It seems necessary, however, to acquire better knowledge of those factors affecting the structure and function of the auditory system when electrical stimulation is used.
In an effort to understand the influence of acoustic stimulation on the developing brain, there have been experimental manipulations on the end organs to deprive the system of input. Deafening has been produced by surgical or pharmacologic manipulations of the auditory periphery as well as by congenital or hereditary defects. Each form of deafness is accompanied by its own potential complication. One must be vigilant for indirect surgical trauma, nonspecific drug effects, and downstream genetic influences.
Models of Deafness and the Cochlear Nucleus
The least invasive manipulation on the auditory system is produced by the introduction of conductive hearing loss. This method basically involves the “plugging” of the ear canal with a malleable substance or removing one of the ossicles. Results from ear plugging have been inconsistent, probably because of the high rates of spontaneous discharges in auditory nerve fibers, which are not affected by this manipulation.92 Even with extreme plugging strategies, the effects are relatively small and show signs of recovery.93–95
Surgical ablation of the cochleae in neonatal animals produces a profound SNHL and results in ˜50% ipsilateral volume loss, 30 to 40% cell body shrinkage, and >50% neural death in the CN.81,96,97 Importantly, the loss of central neurons following cochlear ablation is restricted to a critical period that appears to end at the onset of hearing.98,99 Although deafferentation during cochlear development results in widespread neural loss in the CN, similar cochlear damage after the onset of hearing results in atrophic changes but not neural loss. Given that most forms of SNHL occur following the onset of hearing,100–102 these results imply that the major transneuronal effects of deafness are atrophic, with relatively small amounts of neural loss.
An alternative form of deafening is provided by the administration of ototoxic drugs. These drugs (e.g., amikacin, neomycin, kanamycin, ethacrynatic acid) are known to cause a loss of hair cell receptors and deafness7,103–105 as described above. Following ganglion cell loss, there is a reduction in CN volume and distinct cell shrinkage in cats, gerbils, and guinea pigs,6,106–108 with the effect on the ventral CN much greater compared with that on the dorsal CN. This form of deafening represents the model of choice for current investigations of this topic.
The congenitally deaf white cat (DWC) represents a third alternative for these kinds of studies.19,20,82,109–114 DWCs have long been of interest because an autosomal dominant gene links coat color, eye pigment, and deafness.115–119 This well-documented syndrome is probably a variably penetrant and variably expressive syndrome affecting several characteristics including cochlear pathology120 that was first described in congenitally deaf patients.121,122 The pathology in the DWC ranges from moderate hair cell loss to complete collapse of the organ of Corti with corresponding degrees of hearing loss.19,20,110,123–125 For those animals with a profound SNHL, deafness is manifest by the collapse of Reissner’s membrane onto the undifferentiated organ of Corti, thinning of the stria vascularis, and malformation of the tectorial membrane. A variable degree of SGN degeneration proceeds over time,118,124 and distinct atrophic features are evident in the CN including a 50% reduction in nuclear volume and a differential shrinkage of separate cell types.20,109,126,127 Furthermore, a hypertrophy of synapses is seen on some but not other CN neurons.82 Spherical and globular bushy cells represent two major cell classes in the ventral CN. Spherical bushy cells receive large axosomatic endings called endbulbs of Held, whereas globular bushy cells received smaller endbulbs. Congenital deafness results in a reduction in endbulb branching, reduction of presynaptic vesicles, flattening and hypertrophy of postsynaptic densities, and loss of intermembranous channels. Synapses on spherical bushy cells are severely affected by a SNHL, whereas those on globular bushy cells are less so. In contrast, synapses on multipolar cells exhibit only slight structural abnormalities82,113,128 (Fig. 3–5).
The structural abnormalities in CN neurons in response to a SNHL seem a natural consequence of abnormal inputs from the auditory nerve. Congenital and ototoxic deafness significantly reduces the levels of spontaneous activity in auditory nerve fibers of the cat.17,20 Variations in spontaneous activity in auditory nerve fibers have an effect on spherical bushy cells even in normal hearing cats.129 Deafness-induced changes are expressed as increased thresholds to activation,14 smaller action potentials, smaller after-hyperpolarizations, and shorter membrane time constants.130
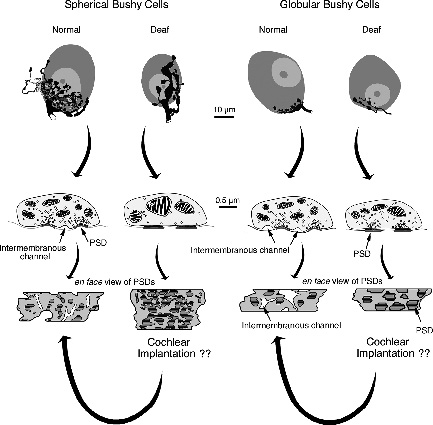
Effects of Electrical Stimulation
The question naturally arises as to whether electrical stimulation of the auditory nerve can effectively prevent, ameliorate, or reverse the deleterious effects of deafness. Inherent to the interpretation of implantation and stimulation results is a consideration that either or both manipulations can contribute to changes additional to those caused by SNHL, and the nature of these changes is not necessarily predictable. Nevertheless, given the notion of a “critical period” in auditory system development, deafened animals are being used to develop experimental models. Furthermore, children, especially prelingually deaf children, are postulated to receive additional benefit from early electrical stimulation via cochlear implants. The rationale is that the preservation of neurons and synapses represents the substrate for improved development of language skills.
The results of electrical stimulation of the auditory nerve on CN structure have not been uniform. In deafened guinea pigs, chronic electrical stimulation of one cochlea resulted in an increase in the size of octopus cells in the ipsilateral CN compared with those on the contralateral side.131 If specific cell types were not considered, analysis of all neurons suggested that there was no effect of electrical stimulation. Likewise, there was no statistically significant difference in nuclear volume between the ipsilateral and contralateral sides. In a similar study using unilateral electrical stimulation of ototoxically deafened cats, the size of spherical bushy cells was reduced 20% by deafening, but stimulation did not produce any difference between the ipsilateral and contralateral sides.132 In related studies, these same comparisons revealed a small (6%) but statistically significant increase in somatic cross-sectional area between ipsilateral and contralateral spherical bushy cells.106,133 There were also mixed results regarding difference in volumes of the cochlear nuclei and somatic sizes of various cell types in the stimulated and non-stimulated sides. Finally, no work has yet examined the effects of chronic electrical stimulation of the auditory nerve on ultrastructural changes to endbulb synapses on spherical or globular bushy cells in the anteroventral CN (Fig. 3–5).
The conclusion from these observations is that electrical stimulation of the cochlea in deaf animals exerts, at best, a small increase in cell body size for CN neurons. There are, however, several considerations that temper this interpretation. First, it isn’t always clear that the cells sampled were those that were stimulated. Because the stimulating electrodes are placed in the basal (high frequency) end of the cochlea, the sampling must also occur in the high-frequency regions of the nuclei. In several studies the sampling was from spherical busy cells in the rostral pole of the anteroventral CN, and this region is known to be responsive primarily to low frequencies.134 Second, using the contralateral CN as a control may not be appropriate with evidence of strong commissural connections between cochlear nuclei.135–139 Stimulation of the ipsilateral CN by auditory nerve fibers undoubtedly produces activity in the contralateral CN via the commissural projections, thereby negating the idea that there is a simple dichotomy produced by the manipulation.
What emerges from these studies is the conclusion that congenital deafness, regardless of its cause, results in transneuronal changes that are expressed at the level of the CN. We shall now examine deafness-induced effects at higher centers of the auditory system. Ascending projections out of the CN exhibit abnormal pathways97,140–142 that impact on the structure of the target brainstem nuclei, including the superior olivary nuclei109,111,143 and inferior colliculus.144–147 These wide-ranging abnormalities are implicated as part of the explanation of why cochlear implants do not always produce beneficial outcomes.
♦ Higher Auditory Centers
The variable synaptic abnormalities within the CN in response to an SNHL, summarized in Fig. 3–5, may contribute to differential transmission delays along the central pathways, leading to corrupted temporal processing of auditory information initiated by cochlear implants. Changes within the CN could obviously have widespread effects because this structure serves as the gateway to the central auditory system. As one ascends the auditory pathway, it seems that transneuronal changes can occur at all levels.
Deafness and the Superior Olivary Complex
The deleterious effects of deafness evident within the CN, are also seen in more central structures. In the DWC, microscopic analysis of the lateral and medial superior olive and the medial nucleus of the trapezoid body demonstrated that neural soma and nuclei areas were 33 to 50% smaller in deaf compared with hearing cats.109 The size of cerebellar Purkinje cells was not affected by an SNHL. There was also a 35% decrease in the number of synapses per unit somatic length in these nuclei in DWCs.111 Analysis of the medial superior olive from deaf humans suggests a 20 to 30% reduction in neural soma area for the denervated structure.88
Deafness and the Inferior Colliculus
In the midbrain, the central nucleus of the inferior colliculus (ICC) represents a key structure in the central auditory pathway. It is tonotopically organized and receives strong excitatory input from the contralateral ear, although most ICC neurons are binaurally influenced. Inputs to the ICC arise bilaterally from the superior olivary complex via the lateral lemniscus (decussating fibers represent the major pathway) and contralaterally from the dorsal and ventral cochlear nuclei.148,149
Following a SNHL, significant structural abnormalities are observed in unilaterally deaf young animals when compared with both normal hearing and bilaterally deafened animals. Such studies revealed an increase in the afferent projection from the normal CN to the ipsilateral ICC.140,141,150 In contrast, bilaterally deaf animals show symmetry of the ascending projection that is similar to that in normal hearing animals.112,151 It would appear that these changes are driven by asymmetrical input to the central auditory system as a result of unilateral deafness. It is worth noting that the great majority of cochlear implant subjects also receive a strongly asymmetrical input via a single cochlear implant.
No difference was found between hearing and unilaterally deaf animals when studying ICC ultrastructure after 1 year of chemically induced deafness, although in bilaterally deaf cats, there was a reduction in the number of synapses compared with partial hearing or normal hearing cats.144 A small but statistically significant reduction in neuronal soma area may be present in bilaterally but not unilaterally deaf cats.146
Anatomic Changes Due to Electrical Stimulation
In patients who had some exposure to electrical stimulation via a cochlear implant, the range of neuronal soma size in the ICC was found to be as much as 30% smaller than normal.88 Small study size, sampling bias, and laterality of stimulation may have obscured trophic effects if they were present, because all cell area averages were smaller than normal hearing controls. Cell soma area was smaller in cases of cochlear pathology than in cases of auditory nerve sectioning. This finding was postulated to be due to a dystrophic effect on neurons from a diseased cochlea, rather than the loss of trophic activity. This inference may have implications for models of deafness based on cochlear abnormalities. Alternatively, neuronal soma size may not be a relevant (or is at best a crude) parameter in evaluating CNS changes due to deafness and electrical stimulation.
Metabolic Changes Due to Electrical Stimulation
Metabolic markers such as 2-deoxyglucose (2-DG) have been used to study the extent of neural activation in the ICC in response to electrical stimulation of the auditory nerve. Brown et al152 compared the effects of electrical versus auditory stimulation on 2-DG uptake in cats. Using acute, unilaterally deafened animals, the 2-DG uptake that was evoked from the deafened, electrically stimulated cochlea resembled that seen in the acoustically stimulated contralateral ICC. The location and extent of 2-DG uptake was dependent on the electrode configuration, electrode location, and stimulus intensity, although any increase in 2-DG uptake may also reflect an additive component arising from the ipsilateral auditory stimulation. In a different set of experiments, bilaterally deafened guinea pigs did not exhibit significantly reduced 2-DG activity in the ICC, and remained responsive to acute electrical stimulation after 15 months of deafness.153 Schwartz et al154 similarly observed normal 2-DG uptake in electrically stimulated deaf guinea pigs. The 2-DG labeling equaled but did not surpass levels observed in acoustically stimulated normal hearing controls. This effect was seen at increasing levels of stimulation, and with chronic stimulation. Collectively these studies suggest that in the unstimulated state, basic pathways for auditory information to the ICC in deafened animals remain largely functionally and structurally intact, indicating a powerful role for genetic programming of auditory connections.
Physiologic Changes Due to Electrical Stimulation
Frequency Representation in the Inferior Colliculus
Single cell activity evoked by electrical stimulation of the auditory nerve in neonatally deafened cats has revealed that major physiologic features of the ICC are preserved over wide durations of deafness.145 The spatial tuning of the ICC studied in these acutely stimulated cats revealed that long-term deafness reduced the distance between basal and apical electrode neural representation in the ICC compared with that seen in cats with more auditory experience.
Experiments using neonatally deafened, chronically electrically stimulated cats have also been performed. Snyder et al155 observed an increase in the ICC neural representation of the electrically stimulated sectors of auditory nerve. Correlating ICC recording depth with neural activity demonstrated a significant increase in neural representation of that sector of auditory nerve subject to chronic stimulation. Moreover, chronic electrical stimulation of the auditory nerve can induce a similar degree of plastic expansion in the ICC of adult-deafened implanted cats.156 More recently, ICC recordings using multielectrode arrays have been implemented.157 When applied chronically, this technique promises to provide a longitudinal view of neural activity across the ICC in response to cochlear implantation.
Alternating stimulation of separate areas of the auditory nerve may preserve, or perhaps even sharpen, ICC representation of those stimulated sectors of auditory nerve.158 These studies indicate that even in prolonged early-onset deafness, basic cochleotopic and neural representation of electrical stimuli may be elicited in the ICC. This developmental feature, however, is clearly modifiable by sensory experience. Neural plasticity of the ICC seems to expand the neural representation of chronically stimulated sectors of auditory nerve, even when those sectors receive stimulation long after the onset of deafness.
Temporal Response Properties
The ability of neurons in the ICC to synchronize their spike discharges to an auditory stimulus is referred to as temporal response properties. These properties allow for the encoding of sound features for speech recognition and word discrimination. Properties such as latency, jitter, and spike rate have been studied. Data are collected by presenting different electrical stimuli to deaf implanted animals, and modifying the duration of deafness, the duration of electrical stimulation, or the stimulus characteristics. Evidence suggests that early-onset deafness followed by chronic electrical stimulation of the auditory nerve may enhance the capacity of the ICC to encode high pulse frequencies, beyond those seen in normal hearing animals.159 In addition, there is a maximum electrical pulse rate that may be encoded by the ICC, findings that have implications for stimulus parameters in the application of cochlear implants.145,159 Neurons in the ICC also appear to have higher temporal resolution when stimulated using amplitude-modulated electrical pulses compared with unmodulated stimuli.160 Most ICC neurons are able to encode frequency-modulated stimuli up to 40 Hz. The carrier pulse rate eliciting response of neurons in the ICC was 104 pulses per second in unmodulated stimuli. By amplitude-modulating the stimuli, carrier pulse rate could exceed 600 pulses per second. Distortion of the neural response was observed as the ratio of modulation to carrier rate approached one fourth to one sixth. This observation may be significant, because cochlear implants can exceed these parameters. Following chronic stimulation at high rates, the ICC seems able to increase its average maximum following-frequency.161 High stimulation rates do not appear to impact the peak maximum following-frequency.
Increased latency and jitter of single unit responses were seen in long-term deaf, acutely stimulated cats.145 Elevated stimulus levels ameliorated these changes. Significantly, chronically stimulating deaf cats at high rates reduced the median latency below that seen in normal-hearing cats.161 Overall, the ICC appears to retain the basic neural properties exhibited by normal-hearing animals. Chronic electrical stimulation may increase the temporal resolving capacity of the ICC, but the significance of this observation remains unknown.
♦ The Auditory Cortex
In 1942 Woolsey and Walzl162 reported the first cortical responses to electrical stimulation of the auditory nerve in normal-hearing cats. Subsequently, the primary auditory cortex (AI) in the normal-hearing animal was shown to be cochleotopically organized, and individual neurons within layer III/IV have well-characterized input-output functions for electrical stimulation and decreasing response latencies (to a minimum around 8 milliseconds) with increasing stimulus strength.163–166
The changes in the auditory cortex after a SNHL are dependent on many factors, including the severity of the SNHL, whether the SNHL is unilateral or bilateral, and the developmental stage at the time of the SNHL. A further complication in the interpretation of changes in the auditory cortex in response to a SNHL, is that many “downstream” changes occur as a result of the SNHL (see previous sections), which effect the input, and the organization of that input, into the auditory cortex. At least some of the changes that occur in the auditory cortex as a result of a SNHL are plastic, and the return of input to the auditory cortex, via electrical stimulation of the auditory nerve, results in the reorganization of the auditory cortex. It is the plastic nature of the auditory cortex that is likely to be an underlying factor in the continued improvement in performance of cochlear implant patients with device use.167
Morphological Changes Following Deafness
In contrast to the lower auditory centers, there is a scarcity of data on the morphologic changes that occur in the auditory cortex as a result of a long-term SNHL.168 Nonetheless, reports of research in rabbits that were unilaterally deafened as neonates indicate that there is no change in neuron soma area, number of dendritic branches, or total basal dendritic length.169 However, there is an apparent reduction in the number of spines along basal dendrites of lamina III/IV pyramidal neurons of the auditory cortex,170 and a reorganization of “spine-free” but not “spiny” dendrites, with an increase in “nonspiny” dendrite length mostly in a tangential direction.171 In combination, these results indicate a loss of synapses within the auditory cortex after a prolonged deprivation of auditory input during development.
Although there have been reports that after pure tone stimulation the location of c-FOS–positive neurons within the auditory cortex (c-FOS is a protein associated with learning172) matched the expected tonotopic organization,173 there have been no reports of the effects of SNHL on either its localization or expression.
Physiologic Changes Following Deafness
The motto “use it or lose it” is often apt for cortical centers, and so reports of the activation of the auditory cortex by other modalities after an early SNHL are not surprising.174–176,176 However, as is the case for the ICC, there is evidence of the development of a “rudimentary cochleotopic representation” within AI, even when completely deprived of input.168,177 Other “higher-order” cortical auditory centers, including the secondary auditory cortex, on the other hand, appear to be more susceptible to “infiltration” from other modalities and exhibit more plasticity than AI in the cat.178,179
Field potentials and single-and multiunit recordings from layers III/IV of the auditory cortex in the DWC have shown that not only is a rudimentary cochleotopic representation present, but basic response properties, including growth functions and latencies, were similar to those in normal-hearing cats.177 Corroborating results have been reported for short-term neonatally deafened cats,180 although the cochleotopic organization was less robust, particularly in the dorsal part of AI, and became further degraded throughout the entire AI with extended periods of deafness.
Clearly, the AI does not develop entirely normally in the absence of sensory input; in fact there are different changes in the development of different layers within AI. Although the DWC develops a rudimentary cochleotopic representation in layers III/IV, there is a decrease in current sinks (and, therefore, presumably synaptic currents) at long (>30 milliseconds) latencies in layers II, III, and IV.181 The decrease in current sinks is even more evident in the deeper (infragranular) layers IV, V, and VI, and in these deeper layers the decrease is evident at all latencies. The infragranular layers are considered to be the output layers of AI, and therefore, in the DWC “efferent projections of the primary auditory cortex to sub-cortical structures and possibly also to higher-order cortical areas are probably substantially diminished.”182
The effects of a restricted unilateral SNHL in adult animals, resulting from a cochlea lesion, and subsequent exposure to at least some auditory input, rather than a complete bilateral SNHL, are apparent in the reorganization of the contralateral AI. Under such conditions, the normal cochleotopic organization of the contralateral AI is distorted, and acoustic frequencies that correspond to regions of cochlea near the lesion-edge become grossly overrepresented.183–186A unilateral SNHL not only affects the contralateral AI, but also results in the ipsilateral AI becoming more sensitive.185,187–189 Such reorganization could be due to “rapid disinhibition or true plastic reorganization with morphological changes,”182 and a contribution from the reorganization of interhemispheric corticocortical projections cannot be excluded.
Many of the studies of SNHL-induced changes in the auditory cortex utilize congenitally or neonatally deafened animal models, which allow access to the early critical period in development. However, changes in the response of neurons within the auditory cortex to a SNHL are not limited to this critical period, but can occur later in development.189 There is a growing body of work supporting the plasticity of the adult auditory cortex, including AI.190–192
Response to Chronic Electrical Stimulation
Chronic, behaviorally relevant, intracochlear electrical stimulation results in plastic changes in the deaf auditory cortex. Compared with unstimulated deaf controls, cats subjected to chronic electrical stimulation exhibit an increase in long-latency (>150 milliseconds) field potentials, larger current source densities (particularly in layers II and III) and more sustained single-and multiunit activity.193 There is also an expansion in the area of cortex activated by the electrical stimulation of the auditory nerve.193 The resulting response properties of AI in chronically stimulated deaf cats are similar to those reported in normal-hearing animals. The long-latency responses are thought to be mediated by corticothalamic loops, and are proposed to be essential for short-term memory and processing in higher-order auditory centers.182 Therefore, behaviorally relevant, chronic intracochlear electrical stimulation appears to allow the auditory cortex to achieve an experience-dependent maturation, albeit not precisely as it would have occurred in the absence of a SNHL.
Clinical Changes Following Deafness
Many of the reports of changes in the auditory cortex in deaf patients, as in the animal studies of the cortex, have been focused on the “use it or lose it” scenario.194 Therefore, it comes as no surprise that it has been reported that in pre-lingual deaf patients “the brain region usually reserved for hearing may be activated by other sensory modalities.”195 However, the auditory deprivation–induced “takeover” of auditory areas appears to be limited to secondary auditory areas (supratemporal gyrus/perisylvian region), which are normally used for auditory processing and language.195–197
Consistent with results reported from the animal models, the connections to AI appear to be more robustly organized than those to the secondary auditory areas, as there is no evidence of cross-modal activation of AI using either positron emission tomography (PET)198 or functional magnetic resonance imaging (fMRI).199 Auditory evoked potentials recorded from both cochlear implant and normal hearing subjects also suggest near-normal maturation of middle (IV and deep III) cortical layers. However, there appears to be altered maturation or input to the superficial (II, upper III) layers,200 particularly in the absence of auditory input during the early critical period.201,202 Therefore, as in the animal models, it appears that the auditory cortex, and in particular AI, remains in an immature state in prelingual deaf patients.203
Ipsilateral-contralateral differences in the responses of the auditory cortex in unilaterally deaf subjects are smaller than in normal-hearing subjects [magnetoencephalogram (MEG)204–207 and fMRI208]. The ipsilateral-contralateral changes can also occur in adult subjects as a result of a late-onset unilateral hearing loss,209 again highlighting the plasticity of the auditory system in the adult brain. Some changes may manifest within weeks of the hearing loss,210 but changes continue to occur over a prolonged time course of 2 years or more,209 suggesting there may be both an unmasking of ordinarily silent inputs and morphologic changes. Interestingly, changes in the response of the ipsilateral auditory cortex may also be dependent on which side the unilateral deafness occurred,211 indicating that these changes may be at least partially a result of changes peripheral to the auditory cortex. These results emphasize the difficulties in determining if functional changes in the response of the auditory cortex are the result of changes within the cortex itself, or a result of downstream changes.
Despite many years of auditory deprivation, during which very low levels of activity are evident in AI, its activation can be seen using a variety of imaging techniques after the return of auditory input via a cochlear implant.212–215 In fact, after long-term cochlear implant use, auditory evoked potentials recorded from prelingual deaf patients, implanted in early childhood, appear nearly normal, suggesting an activation-induced maturation of the auditory cortex.216 There are also plastic changes within the “higher-order” auditory centers, with the amount of activity in these centers in pre-lingual deaf patients with a cochlear implant decreasing with experience in using the implant.217 There also is less activity seen in the higher-order auditory centers in prelingual deaf cochlear implant patients than in postlingual deaf cochlear implant patients.218 Finally, long-term cochlear implant use also appears to enhance “top-down” feedback modulation of processing within lower auditory centers.194
A final interesting observation is that there is a positive correlation between speech perception and low resting activity in AI prior to cochlear implantation for the prelingual deaf.217 This relationship suggests that although AI and other higher-order auditory centers are capable of plastic change, as evident in the continued improvement in auditory performance with increasing experience using a cochlear implant,167 the best clinical outcomes for cochlear implant patients may in fact occur with the most immature auditory cortex, or the “cleanest sheet.”
♦ Conclusions
Auditory experience plays a key role in laying down the fine organizational structure of the auditory pathway onto a framework generated by genetic cues. A lack of auditory experience during development results in a more rudimentary pathway; however, this reduced level of organization appears sufficient to provide both the temporal and spatial cues necessary for speech perception using a cochlear implant. Importantly, the ability of this pathway to undergo plastic reorganization following cochlear implantation is now recognized as a major factor underlying the clinical success of these devices. This chapter reviewed the degenerative and atrophic changes that occur within the auditory pathway following a SNHL and the trophic and functional reorganization that occurs following reafferentation via a cochlear implant.
Acknowledgments
This work was funded by the National Institutes of Health–National Institute on Deafness and Other Communication Disorders (NIDCD) (DC00232 and DC00027 and NO1-DC-3–1005), the Wagstaff Fellowship from the Royal Victorian Eye and Ear Hospital, and the Bionic Ear Institute. Sarah McGuiness, Rodney Millard, Stephanie Epp, and Anne Coco made important contributions to this work, and Dexter Irvine provided critical comment on an earlier version of this chapter, for which we are most grateful.
References
1. Grill WM. Electrical stimulation of the peripheral nervous system: biophysics and excitation properties. In: Horch KW, Dhillon GS, eds. Neuroprosthetics: Theory and Practice, vol 2. Singapore: World Scientific Publishing, 2004:319–341
2. Gantz BJ, Woodworth GG, Knutson JF, Abbas PJ, Tyler RS. Multivariate predictors of audiological success with multichannel cochlear implants. Ann Otol Rhinol Laryngol 1993;102:909–916
3. Nadol JB Jr, Young YS, Glynn RJ. Survival of spiral ganglion cells in profound sensorineural hearing loss: implications for cochlear implantation. Ann Otol Rhinol Laryngol 1989;98:411–416
4. Terayama Y, Kaneko Y, Kawamoto K, Sakai N. Ultrastructural changes of the nerve elements following disruption of the organ of Corti. I. Nerve elements in the organ of Corti. Acta Otolaryngol 1977;83:291–302
5. Spoendlin H. Factors inducing retrograde degeneration of the cochlear nerve. Ann Otol Rhinol Laryngol Suppl 1984;112:76–82
6. Hardie NA, Shepherd RK. Sensorineural hearing loss during development: morphological and physiological response of the cochlea and auditory brainstem. Hear Res 1999;128:147–165
7. Leake PA, Hradek GT. Cochlear pathology of long term neomycin induced deafness in cats. Hear Res 1988;33:11–33
8. Shepherd RK, Hardie NA. Deafness induced changes in the auditory pathway: implications for cochlear implants. Audiol Neurootol 2001;6:305–318
9. Spoendlin H, Schrott A. Analysis of the human auditory nerve. Hear Res 1989;43:25–38
10. Elverland HH, Mair IW. Hereditary deafness in the cat. An electron microscopic study of the spiral ganglion. Acta Otolaryngol 1980;90:360–369
11. Nadol JB Jr. Degeneration of cochlear neurons as seen in the spiral ganglion of man. Hear Res 1990;49:141–154
12. Steel KP, Bock GR. Electrically-evoked responses in animals with progressive spiral ganglion degeneration. Hear Res 1984;15: 59–67
13. McGuinness SL, Shepherd RK. Exogenous BDNF rescues rat spiral ganglion neurons in vivo. Otol Neurotol 2005;26:1064–1072
14. Shepherd RK, Roberts LA, Paolini AG. Long-term sensorineural hearing loss induces functional changes in the rat auditory nerve. Eur J Neurosci 2004;20:3131–3140
15. Takeno S, Wake M, Mount RJ, Harrison RV. Degeneration of spiral ganglion cells in the chinchilla after inner hair cell loss induced by carboplatin. Audiol Neurootol 1998;3:281–290
16. Gillespie LN, Clark GM, Marzella PL. Delayed neurotrophin treatment supports auditory neuron survival in deaf guinea pigs. Neuroreport 2004;15:1121–1125
17. Shepherd RK, Javel E. Electrical stimulation of the auditory nerve. I. Correlation of physiological responses with cochlear status. Hear Res 1997;108:112–144
18. Heid S, Hartmann R, Klinke R. A model for prelingual deafness, the congenitally deaf white cat–population statistics and degenerative changes. Hear Res 1998;115:101–112
19. Mair IW. Hereditary deafness in the white cat. Acta Otolaryngol Suppl 1973;314:1–48
20. Ryugo DK, Rosenbaum BT, Kim PJ, Niparko JK, Saada AA. Single unit recordings in the auditory nerve of congenitally deaf white cats: morphological correlates in the cochlea and cochlear nucleus. J Comp Neurol 1998;397:532–548
21. Liberman MC, Kiang NY. Acoustic trauma in cats. Cochlear pathology and auditory-nerve activity. Acta Otolaryngol Suppl 1978;358:1–63
22. Johnsson LG. Sequence of degeneration of Corti’s organ and its first-order neurons. Ann Otol Rhinol Laryngol 1974;83:294–303
23. Suzuka Y, Schuknecht HF. Retrograde cochlear neuronal degeneration in human subjects. Acta Otolaryngol Suppl 1988;450:1–20
24. Imamura S, Adams JC. Distribution of gentamicin in the guinea pig inner ear after local or systemic application. J Assoc Res Otolaryngol 2003;4:176–195
25. Ylikoski J, Pirvola U, Moshnyakov M, Palgi J, Arumae U, Saarma M. Expression patterns of neurotrophin and their receptor mRNAs in the rat inner ear. Hear Res 1993;65:69–78
26. Schecterson LC, Bothwell M. Neurotrophin and neurotrophin receptor mRNA expression in developing inner ear. Hear Res 1994;73:92–100
27. Fritzsch B, Barbacid M, Silos-Santiago I. Nerve dependency of developing and mature sensory receptor cells. Ann N Y Acad Sci 1998;855:14–27
28. Ernfors P, Van De Water T, Loring J, Jaenisch R. Complementary roles of BDNF and NT-3 in vestibular and auditory development. Neuron 1995;14:1153–1164
29. Stankovic K, Rio C, Xia A, et al. Survival of adult spiral ganglion neurons requires erbB receptor signaling in the inner ear. J Neurosci 2004;24:8651–8661
30. Hinojosa R, Marion M. Histopathology of profound sensorineural deafness. Ann N Y Acad Sci 1983;405:459–484
31. Nadol JB Jr. Patterns of neural degeneration in the human cochlea and auditory nerve: implications for cochlear implantation. Otolaryngol Head Neck Surg 1997;117(3 pt 1):220–228
32. Otte J, Schunknecht HF, Kerr AG. Ganglion cell populations in normal and pathological human cochleae. Implications for cochlear implantation. Laryngoscope 1978;88(8 pt 1):1231–1246
33. Felix H, Pollak A, Gleeson M, Johnsson LG. Degeneration pattern of human first-order cochlear neurons. Adv Otorhinolaryngol 2002;59:116–123
34. Miura M, Sando I, Hirsch BE, Orita Y. Analysis of spiral ganglion cell populations in children with normal and pathological ears. Ann Otol Rhinol Laryngol 2002;111(12 pt 1):1059–1065
35. Waltzman SB, Cohen NL, Green J, Roland JT Jr. Long-term effects of cochlear implants in children. Otolaryngol Head Neck Surg 2002;126:505–511
36. Javel E, Shepherd RK. Electrical stimulation of the auditory nerve. III. Response initiation sites and temporal fine structure. Hear Res 2000;140:45–76
37. Hartmann R, Topp G, Klinke R. Discharge patterns of cat primary auditory fibers with electrical stimulation of the cochlea. Hear Res 1984;13:47–62
38. van den Honert C, Stypulkowski PH. Physiological properties of the electrically stimulated auditory nerve. II. Single fiber recordings. Hear Res 1984;14:225–243
39. Frijns JH, de Snoo SL, ten Kate JH. Spatial selectivity in a rotationally symmetric model of the electrically stimulated cochlea. Hear Res 1996;95:33–48
40. Tasaki I. New measurements of the capacity and the resistance of the myelin sheath and the nodal membrane of the isolated frog nerve fiber. Am J Physiol 1955;181:639–650
41. Koles ZJ, Rasminsky M. A computer simulation of conduction in demyelinated nerve fibres. J Physiol 1972;227:351–364
42. Zhou R, Abbas PJ, Assouline JG. Electrically evoked auditory brainstem response in peripherally myelin-deficient mice. Hear Res 1995;88:98–106
43. Araki S, Kawano A, Seldon L, Shepherd RK, Funasaka S, Clark GM. Effects of chronic electrical stimulation on spiral ganglion neuron survival and size in deafened kittens. Laryngoscope 1998;108:687–695
44. Xu J, Shepherd RK, Millard RE, Clark GM. Chronic electrical stimulation of the auditory nerve at high stimulus rates: a physiological and histopathological study. Hear Res 1997;105:1–29
45. Shepherd RK, Matsushima J, Martin RL, Clark GM. Cochlear pathology following chronic electrical stimulation of the auditory nerve: II. Deafened kittens. Hear Res 1994;81:150–166
46. Ni D, Shepherd RK, Seldon HL, Xu S-A, Clark GM, Millard RE. Cochlear pathology following chronic electrical stimulation of the auditory nerve. I: normal hearing kittens. Hear Res 1992;62:63–81
47. Shepherd RK, Clark GM, Black RC. Chronic electrical stimulation of the auditory nerve in cats. Physiological and histopathological results. Acta Otolaryngol Suppl 1983;399:19–31
48. Leake-Jones PA, Rebscher SJ. Cochlear pathology with chronically implanted scala tympani electrodes. Ann N Y Acad Sci 1983;405:203–223
49. Walsh SM, Leake-Jones PA. Chronic electrical stimulation of auditory nerve in cat: physiological and histological results. Hear Res 1982;7:281–304
50. Brummer SB, Turner MJ. Electrochemical considerations for safe electrical stimulation of the nervous system with platinum electrodes. IEEE Trans Biomed Eng 1977;24:59–63
51. Robblee LS, McHardy J, Agnew WF, Bullara LA. Electrical stimulation with Pt electrodes. VII. Dissolution of Pt electrodes during electrical stimulation of the cat cerebral cortex. J Neurosci Methods 1983;9:301–308
52. Rose TL, Robblee LS. Electrical stimulation with Pt electrodes. VIII. Electrochemically safe charge injection limits with 0.2 ms pulses. IEEE Trans Biomed Eng 1990;37:1118–1120
53. Shepherd RK, Linahan N, Xu J, Clark GM, Araki S. Chronic electrical stimulation of the auditory nerve using non-charge-balanced stimuli. Acta Otolaryngol 1999;119:674–684
54. Shepherd RK, Matsushima J, Millard RE, Clark GM. Cochlear pathology following chronic electrical stimulation using non-charge balanced stimuli. Acta Otolaryngol 1991;111:848–860
55. Huang CQ, Carter PM, Shepherd RK. Stimulus induced pH changes in cochlear implants: an in vitro and in vivo study. Ann Biomed Eng 2001;29:791–802
56. Huang CQ, Shepherd RK, Carter PM, Seligman PM, Tabor B. Electrical stimulation of the auditory nerve: direct current measurement in vivo. IEEE Trans Biomed Eng 1999;46:461–470
57. Hegarty JL, Kay AR, Green SH. Trophic support of cultured spiral ganglion neurons by depolarization exceeds and is additive with that by neurotrophins or cAMP and requires elevation of [Ca2+]i within a set range. J Neurosci 1997;17:1959–1970
58. Hansen MR, Zha XM, Bok J, Green SH. Multiple distinct signal pathways, including an autocrine neurotrophic mechanism, contribute to the survival-promoting effect of depolarization on spiral ganglion neurons in vitro. J Neurosci 2001;21:2256–2267
59. Lousteau RJ. Increased spiral ganglion cell survival in electrically stimulated, deafened guinea pig cochleae. Laryngoscope 1987;97: 836–842
60. Hartshorn DO, Miller JM, Altschuler RA. Protective effect of electrical stimulation in the deafened guinea pig cochlea. Otolaryngol Head Neck Surg 1991;104:311–319
61. Miller CA, Faulkner MJ, Pfingst BE. Functional responses from guinea pigs with cochlear implants. II. Changes in electrophysiological and psychophysical measures over time. Hear Res 1995;92:100–111
62. Mitchell A, Miller JM, Finger PA, Heller JW, Raphael Y, Altschuler RA. Effects of chronic high-rate electrical stimulation on the cochlea and eighth nerve in the deafened guinea pig. Hear Res 1997;105: 30–43
63. Kanzaki S, Stover T, Kawamoto K, et al. Glial cell line-derived neurotrophic factor and chronic electrical stimulation prevent VIII cranial nerve degeneration following denervation. J Comp Neurol 2002;454:350–360
64. Leake PA, Hradek GT, Rebscher SJ, Snyder RL. Chronic intracochlear electrical stimulation induces selective survival of spiral ganglion neurons in neonatally deafened cats. Hear Res 1991;54:251–271
65. Leake PA, Snyder RL, Hradek GT, Rebscher SJ. Chronic intracochlear electrical stimulation in neonatally deafened cats: effects of intensity and stimulating electrode location. Hear Res 1992;64:99–117
66. Leake PA, Snyder RL, Hradek GT, Rebscher SJ. Consequences of chronic extracochlear electrical stimulation in neonatally deafened cats. Hear Res 1995;82:65–80
67. Leake PA, Hradek GT, Snyder RL. Chronic electrical stimulation by a cochlear implant promotes survival of spiral ganglion neurons after neonatal deafness. J Comp Neurol 1999;412:543–562
68. Li L, Parkins CW, Webster DB. Does electrical stimulation of deaf cochleae prevent spiral ganglion degeneration? Hear Res 1999;133:27–39
69. Shepherd R, Coco A, Epp S, Crook JM. Chronic depolarization enhances the trophic effects of BDNF in rescuing auditory neurons following a sensorineural hearing loss. J Comp Neurol 2005;486:145–158
70. Fritzsch B, Pirvola U, Ylikoski J. Making and breaking the innervation of the ear: neurotrophic support during ear development and its clinical implications. Cell Tissue Res 1999;295:369–382
71. Lefebvre PP, Weber T, Rigo J-M, Staecker H, Moonen G, Van De Water TR. Peripheral and central target-derived trophic factor(s) effects on auditory neurons. Hear Res 1992;58:185–192
72. Ernfors P, Duan ML, ElShamy WM, Canlon B. Protection of auditory neurons from aminoglycoside toxicity by neurotrophin-3. Nat Med 1996;2:463–467
73. Miller JM, Chi DH, O’Keeffe LJ, Kruszka P, Raphael Y, Altschuler RA. Neurotrophins can enhance spiral ganglion cell survival after inner hair cell loss. Int J Dev Neurosci 1997;15:631–643
74. Staecker H, Kopke R, Malgrange B, Lefebvre P, Van de Water TR. NT-3 and/or BDNF therapy prevents loss of auditory neurons following loss of hair cells. Neuroreport 1996;7:889–894
75. Shinohara T, Bredberg G, Ulfendahl M, et al. Neurotrophic factor intervention restores auditory function in deafened animals. Proc Natl Acad Sci U S A 2002;99:1657–1660
76. Wise AK, Richardson R, Hardman J, Clark G, O’Leary S. Resprouting and survival of guinea pig cochlear neurons in response to the administration of the neurotrophins brain-derived neurotrophic factor and neurotrophin-3. J Comp Neurol 2005;487:147–165
77. Gillespie LN, Clark GM, Bartlett PF, Marzella PL. BDNF-induced survival of auditory neurons in vivo: Cessation of treatment leads to an accelerated loss of survival effects. J Neurosci Res 2003;71:785–790
78. Powell TPS, Erulkar SD. Transneuronal cell degeneration in the auditory relay nuclei of the cat. J Anat 1962;96:249–268
79. Webster DB. A critical period during postnatal auditory development of mice. Int J Pediatr Otorhinolaryngol 1983;6:107–118
80. Webster DB. Conductive hearing loss affects the growth of the cochlear nuclei over an extended period of time. Hear Res 1988;32:185–192
81. Hashisaki GT, Rubel EW. Effects of unilateral cochlea removal on anteroventral cochlear nucleus neurons in developing gerbils. J Comp Neurol 1989;283:5–73
82. Ryugo DK, Pongstaporn T, Huchton DM, Niparko JK. Ultrastructural analysis of primary endings in deaf white cats: morphologic alterations in endbulbs of Held. J Comp Neurol 1997;385:230–244
83. Sininger YS, Doyle KJ, Moore JK. The case for early identification of hearing loss in children. Auditory system development, experimental auditory deprivation, and development of speech perception and hearing. Pediatr Clin North Am 1999;46:1–14
84. Marianowski R, Liao WH, Van Den Abbeele T, et al. Expression of NMDA, AMPA and GABA(A) receptor subunit mRNAs in the rat auditory brainstem. I. Influence of early auditory deprivation. Hear Res 2000;150:1–11
85. Clark GM, Shepherd RK, Franz BK, et al. The histopathology of the human temporal bone and auditory central nervous system following cochlear implantation in a patient. Correlation with psychophysics and speech perception results. Acta Otolaryngol Suppl 1988;448:1–65
86. Seldon HL, Clark GM. Human cochlear nucleus: comparison of Nisslstained neurons from deaf and hearing patients. Brain Res 1991;551:185–194
87. Moore JK, Niparko JK, Miller MR, Linthicum FH. Effect of profound hearing loss on a central auditory nucleus. Am J Otol 1994;15: 588–595
88. Moore JK, Niparko JK, Perazzo LM, Miller MR, Linthicum FH. Effect of adult-onset deafness on the human central auditory system. Ann Otol Rhinol Laryngol 1997;106:385–390
89. Waltzman SB, Cohen NL, Shapiro WH. Effects of chronic electrical stimulation on patients using a cochlear prosthesis. Otolaryngol Head Neck Surg 1991;105:797–801
90. Niparko JK, Kirk KI, Mellon NK, Robbins AM, Tucci DL, Wilson BS. Cochlear Implants: Principles and Practices. Philadelphia: Lippincott Williams & Wilkins, 2000
91. Sarant JZ, Blamey PJ, Dowell RC, Clark GM, Gibson WP. Variation in speech perception scores among children with cochlear implants. Ear Hear 2001;22:18–28
92. Tucci DL, Rubel EW. Afferent influences on brain stem auditory nuclei of the chicken: effects of conductive and sensorineural hearing loss on n. magnocellularis. J Comp Neurol 1985;238:371–381
93. Hood LJ, Webster DB. Reversible conductive hearing loss in mice. Ann Otol Rhinol Laryngol 1988;97(3 pt 1):281–285
94. Doyle WJ, Webster DB. Neonatal conductive hearing loss does not compromise brainstem auditory function and structure in rhesus monkeys. Hear Res 1991;54:145–151
95. Moore DR, Hine JE, Jiang ZD, Matsuda H, Parsons CH, King AJ. Conductive hearing loss produces a reversible binaural hearing impairment. J Neurosci 1999;19:8704–8711
96. Trune DR. Influence of neonatal cochlear removal on the development of mouse cochlear nucleus: I. Number, size, and density of its neuronal. J Comp Neurol 1982;209:409–424
97. Moore DR, Kowalchuk NE. Auditory brainstem of the ferret: effects of unilateral cochlear lesions on cochlear nucleus volume and projections to the inferior colliculus. J Comp Neurol 1988;272:503–515
98. Tierney TS, Russell FA, Moore DR. Susceptibility of developing cochlear nucleus neurons to deafferentation-induced death abruptly ends just before the onset of hearing. J Comp Neurol 1997;378: 295–306
100. Marot M, Uziel A, Romand R. Ototoxicity of kanamycin in developing rats: relationship with the onset of the auditory function. Hear Res 1980;2:111–113
101. Shepherd RK, Martin RL. Onset of ototoxicity in the cat is related to onset of auditory function. Hear Res 1995;92:131–142
102. O’Leary SJ, Moore DR. Development of cochlear sensitivity to aminoglycoside antibiotics. Ann Otol Rhinol Laryngol 1998;107: 220–226
103. Hawkins JE Jr. Comparative otopathology: aging, noise, and ototoxic drugs. Adv Otorhinolaryngol 1973;20:125–141
104. Russell NJ, Fox KE, Brummett RE. Ototoxic effects of the interaction between kanamycin and ethacrynic acid. Cochlear ultrastructure correlated with cochlear potentials and kanamycin levels. Acta Otolaryngol 1979;88:369–381
105. Fleckeisen CE, Harrison RV, Mount RJ. Effects of total cochlear haircell loss on integrity of cochlear nucleus. A quantitative study. Acta Otolaryngol Suppl 1991;489:23–31
106. Lustig LR, Leake PA, Snyder RL, Rebscher SJ. Changes in the cat cochlear nucleus following neonatal deafening and chronic intracochlear electrical stimulation. Hear Res 1994;74:29–37
107. Lesperance MM, Helfert RH, Altschuler RA. Deafness induced cell size changes in rostal AVCN of the guinea pig. Hear Res 1995;86:77–81
108. Russell FA, Moore DR. Effects of unilateral cochlear removal on dendrites in the gerbil medial superior olivary nucleus. Eur J Neurosci 1999;11:1379–1390
109. West CD, Harrison JM. Transneuronal cell atrophy in the congenitally deaf white cat. J Comp Neurol 1973;151:377–398
110. Bosher SK, Hallpike CS. Observations on the histological features, development and pathogenesis of the inner ear degeneration of the deaf white cat. Proc R Soc Lond B Biol Sci 1965;162:147–170
111. Schwartz IR, Higa JF. Correlated studies of the ear and brainstem in the deaf white cat: changes in the spiral ganglion and the medial superior olivary nucleus. Acta Otolaryngol 1982;93:9–18
112. Heid S, Jähn-Siebert TK, Klinke R, Hartmann R, Langner G. Afferent projection patterns in the auditory brainstem in normal and congenitally deaf white cats. Hear Res 1997;110:191–199
113. Redd EE, Pongstaporn T, Ryugo DK. The effects of congenital deafness on auditory nerve synapses and globular bushy cells in cats. Hear Res 2000;147:160–174
114. Brown KS, Bergsma DR, Barrow MV. Animal models of pigment and hearing abnormalities in man. Birth Defects Orig Artic Ser 1971;7:102–109
115. Rawitz B. Gehororgan und gehirn eines weissen Hundes mit blauen Augen. Morphol Arbeit 1896;6:545–554
116. Bamber RC. Correlation between white coat colour, blue eyes and deafness in cats. J Genet 1933;27:407–413
117. Wolff D. Three generations of deaf white cats. J Hered 1942;33:39–43
118. Wilson TG, Kane F. Congenital deafness in white cats. Acta Otolaryngol 1959;50:269–275 discussion 275–267
119. Bergsma DR, Brown KS. White fur, blue eyes, and deafness in the domestic cat. J Hered 1971;62:171–185
120. Ryugo DK, Cahill HB, Rose LS, Rosenbaum BT, Schroeder ME, Wright AL. Separate forms of pathology in the cochlea of congenitally deaf white cats. Hear Res 2003;181:73–84
121. Scheibe A. A case of deaf-mutism with auditory atrophy and anomalies of development in the membranous labyrinth of both ears. Arch Otolaryngol 1892;21:12–22
122. Scheibe A. Bildungsanomalien im hautigen labyrinth bei taubstummheit. Z Ohrenheilk 1895;27:95–99
123. Pujol R, Rebillard M, Rebillard G. Primary neural disorders in the deaf white cat cochlea. Acta Otolaryngol 1977;83:59–64
124. Rebillard M, Rebillard G, Pujol R. Variability of the hereditary deafness in the white cat. I. Physiology. Hear Res 1981;5:179–187
125. Rebillard M, Pujol R, Rebillard G. Variability of the hereditary deafness in the white cat. II. Histology. Hear Res 1981;5:189–200
126. Larsen SA, Kirchhoff TM. Anatomical evidence of synaptic plasticity in the cochlear nuclei of white-deaf cats. Exp Neurol 1992;115:151–157
127. Saada AA, Niparko JK, Ryugo DK. Morphological changes in the cochlear nucleus of congenitally deaf white cats. Brain Res 1996;736:315–328
128. Redd EE, Cahill HB, Pongstaporn T, Ryugo DK. The effects of congenital deafness on auditory nerve synapses: type I and type II multipolar cells in the anteroventral cochlear nucleus of cats. J Assoc Res Otolaryngol 2002;3:403–417
129. Ryugo DK, Sento S. Auditory nerve terminals and cochlear nucleus neurons: endbulb of Held and spherical bushy cells. In: Ainsworth WA, ed. Advances in Speech, Hearing and Language Processing. London: Jai Press, 1996:19–40
130. Francis HW, Manis PB. Effects of deafferentation on the electrophysiology of ventral cochlear nucleus neurons. Hear Res 2000;149: 91–105
131. Chouard CH, Meyer B, Josset P, Buche JF. The effect of the acoustic nerve chronic electric stimulation upon the guinea pig cochlear nucleus development. Acta Otolaryngol 1983;95:639–645
132. Hultcrantz M, Snyder R, Rebscher S, Leake P. Effects of neonatal deafening and chronic intracochlear electrical stimulation on the cochlear nucleus in cats. Hear Res 1991;54:272–280
133. Matsushima J-I, Shepherd RK, Seldon HL, Xu S-A, Clark GM. Electrical stimulation of the auditory nerve in deaf kittens: effects on cochlear nucleus morphology. Hear Res 1991;56:133–142
134. Bourk TR, Mielcarz JP, Norris BE. Tonotopic organization of the anteroventral cochlear nucleus of the cat. Hear Res 1981;4:215–241
135. Cant NB, Gaston KC. Pathways connecting the right and left cochlear nuclei. J Comp Neurol 1982;212:313–326
136. Wenthold RJ. Evidence for a glycinergic pathway connecting the two cochlear nuclei: an immunocytochemical and retrograde transport study. Brain Res 1987;415:183–187
137. Shore SE, Godfrey DA, Helfert RH, Altschuler RA, Bledsoe SC Jr. Connections between the cochlear nuclei in guinea pig. Hear Res 1992;62:16–26
138. Schofield BR, Cant NB. Projections from the ventral cochlear nucleus to the inferior colliculus and the contralateral cochlear nucleus in guinea pigs. Hear Res 1996;102:1–14
139. Schofield BR, Cant NB. Origins and targets of commissural connections between the cochlear nuclei in guinea pigs. J Comp Neurol 1996;375:128–146
140. Moore DR, Kitzes LM. Projections from the cochlear nucleus to the inferior colliculus in normal and neonatally cochlea-ablated gerbils. J Comp Neurol 1985;240:180–195
141. Nordeen KW, Killackey HP, Kitzes LM. Ascending projections to the inferior colliculus following unilateral cochlear ablation in the neonatal gerbil, Meriones unguiculatus. J Comp Neurol 1983;214:144–153
142. Russell FA, Moore DR. Afferent reorganisation within the superior olivary complex of the gerbil: development and induction by neonatal, unilateral cochlear removal. J Comp Neurol 1995;352:607–625
143. Jean-Baptiste M, Morest DK. Transneuronal changes of synaptic endings and nuclear chromatin in the trapezoid body following cochlear ablations in cats. J Comp Neurol 1975;162:111–134. http://www3.interscience.wiley.com/cgi-bin/abstract/109685039/ABSTRACT
144. Hardie NA, Martsi-McClintock A, Aitkin L, Shepherd RK. Neonatal sensorineural hearing loss affects synaptic density in the auditory midbrain. Neuroreport 1998;9:2019–2022
145. Shepherd RK, Baxi JH, Hardie NA. Response of inferior colliculus neurons to electrical stimulation of the auditory nerve in neonatally deafened cats. J Neurophysiol 1999;82:1363–1380
146. Nishiyama N, Hardie NA, Shepherd RK. Neonatal sensorineural hearing loss affects neurone size in cat auditory midbrain. Hear Res 2000;140:18–22
147. Vale C, Sanes DH. The effect of bilateral deafness on excitatory and inhibitory synaptic strength in the inferior colliculus. Eur J Neurosci 2002;16:2394–2404
148. Jahn AF, Santos-Sacchi J, eds. Physiology of the Ear. New York: Raven Press, 1988
149. Ehret G. The auditory midbrain, a “shunting-yard” of acoustical information processing. In: Ehret G, Romand R, eds. The Central Auditory System. Oxford: Oxford University Press, 1997:259–316
150. McAlpine D, Martin RL, Mossop JE, Moore DR. Response properties of neurons in the inferior colliculus of the monaurally deafened ferret to acoustic stimulation of the intact ear. J Neurophysiol 1997;78:767–779
151. Moore DR. Auditory brainstem of the ferret: bilateral cochlear lesions in infancy do not affect the number of neurons projecting from the cochlear nucleus to the inferior colliculus. Brain Res Dev Brain Res 1990;54:125–130
153. el-Kashlan HK, Noorily AD, Niparko JK, Miller JM. Metabolic activity of the central auditory structures following prolonged deafferentation. Laryngoscope 1993;103(4 pt 1):399–405
154. Schwartz DR, Schacht J, Miller JM, Frey K, Altschuler RA. Chronic electrical stimulation reverses deafness-related depression of electrically evoked 2-deoxyglucose activity in the guinea pig inferior colliculus. Hear Res 1993;70:243–249
155. Snyder RL, Rebscher SJ, Cao K, Leake PA, Kelly K. Chronic intracochlear electrical stimulation in the neonatally deafened cat. I: Expansion of central representation. Hear Res 1990;50:7–34
156. Moore CM, Vollmer M, Leake PA, Snyder RL, Rebscher SJ. The effects of chronic intracochlear electrical stimulation on inferior colliculus spatial representation in adult deafened cats. Hear Res 2002;164: 82–96
157. Snyder RL, Bierer JA, Middlebrooks JC. Topographic spread of inferior colliculus activation in response to acoustic and intracochlear electric stimulation. J Assoc Res Otolaryngol 2004;5:305–322
158. Leake PA, Snyder RL, Rebscher SJ, Moore CM, Vollmer M. Plasticity in central representations in the inferior colliculus induced by chronic single-vs. two-channel electrical stimulation by a cochlear implant after neonatal deafness. Hear Res 2000;147:221–241
159. Snyder R, Leake P, Rebscher S, Beitel R. Temporal resolution of neurons in cat inferior colliculus to intracochlear electrical stimulation: effects of neonatal deafening and chronic stimulation. J Neurophysiol 1995;73:449–467
160. Snyder RL, Vollmer M, Moore CM, Rebscher SJ, Leake PA, Beitel RE. Responses of inferior colliculus neurons to amplitude-modulated intracochlear electrical pulses in deaf cats. J Neurophysiol 2000;84:166–183
161. Vollmer M, Snyder RL, Leake PA, Beitel RE, Moore CM, Rebscher SJ. Temporal properties of chronic cochlear electrical stimulation determine temporal resolution of neurons in cat inferior colliculus. J Neurophysiol 1999;82:2883–2902
162. Woolsey CN, Walzl EM. Topical projection of nerve fibers from local regions of the cochlea to the cerebral cortex. Bull Johns Hopkins Hosp 1942;71:315–344
163. Popelar J, Hartmann R, Syka J, Klinke R. Middle latency responses to acoustical and electrical stimulation of the cochlea in cats. Hear Res 1995;92:63–77
164. Raggio MW, Schreiner CE. Neuronal responses in cat primary auditory cortex to electrical cochlear stimulation. I. Intensity dependence of firing rate and response latency. J Neurophysiol 1994;72: 2334–2359
165. Schreiner CE, Raggio MW. Neuronal responses in cat primary auditory cortex to electrical cochlear stimulation. II. Repetition rate coding. J Neurophysiol 1996;75:1283–1300
166. Raggio MW, Schreiner CE. Neuronal responses in cat primary auditory cortex to electrical cochlear stimulation: IV. Activation pattern for sinusoidal stimulation. J Neurophysiol 2003;89: 3190–3204
167. Blamey P, Arndt P, Bergeron F, et al. Factors affecting auditory performance of postlinguistically deaf adults using cochlear implants. Audiol Neurootol 1996;1:293–306
168. Shepherd RK, Hartmann R, Heid S, Hardie N, Klinke R. The central auditory system and auditory deprivation: experience with cochlear implants in the congenitally deaf. Acta Otolaryngol Suppl 1997;532: 28–33
169. McMullen NT, Goldberger B, Glaser EM. Postnatal development of lamina III/IV nonpyramidal neurons in rabbit auditory cortex: quantitative and spatial analyses of Golgi-impregnated material. J Comp Neurol 1988;278:139–155
170. McMullen NT, Glaser EM. Auditory cortical responses to neonatal deafening: pyramidal neuron spine loss without changes in growth or orientation. Exp Brain Res 1988;72:195–200
171. McMullen NT, Goldberger B, Suter CM, Glaser EM. Neonatal deafening alters nonpyramidal dendrite orientation in auditory cortex: a computer microscope study in the rabbit. J Comp Neurol 1988;267: 92–106
172. Kandiel A, Chen S, Hillman DE. c-fos gene expression parallels auditory adaptation in the adult rat. Brain Res 1999;839:292–297
173. Zuschratter W, Gass P, Herdegen T, Scheich H. Comparison of frequency-specific c-Fos expression and fluoro-2-deoxyglucose uptake in auditory cortex of gerbils (Meriones unguiculatus). Eur J Neurosci 1995;7:1614–1626
174. Rebillard G, Rebillard M, Pujol R. Factors affecting the recording of visual-evoked potentials from the deaf cat primary auditory cortex (AI). Brain Res 1980;188:252–254
175. Rebillard G, Carlier E, Rebillard M, Pujol R. Enhancement of visual responses on the primary auditory cortex of the cat after an early destruction of cochlear receptors. Brain Res 1977;129:162–164
176. Ahn SH, Oh SH, Lee JS, et al. Changes of 2-deoxyglucose uptake in the rat auditory pathway after bilateral ablation of the cochlea. Hear Res 2004;196:33–38
177. Hartmann R, Shepherd RK, Heid S, Klinke R. Response of the primary auditory cortex to electrical stimulation of the auditory nerve in the congenitally deaf white cat. Hear Res 1997;112:115–133
178. Diamond DM, Weinberger NM. Physiological plasticity of single neurons in auditory cortex of the cat during acquisition of the pupillary conditioned response: II. Secondary field (AII). Behav Neurosci 1984;98:189–210
179. Weinberger NM, Hopkins W, Diamond DM. Physiological plasticity of single neurons in auditory cortex of the cat during acquisition of the pupillary conditioned response: I. Primary field (AI). Behav Neurosci 1984;98:171–188
180. Raggio MW, Schreiner CE. Neuronal responses in cat primary auditory cortex to electrical cochlear stimulation. III. Activation patterns in short-and long-term deafness. J Neurophysiol 1999;82:3506–3526
181. Kral A, Hartmann R, Tillein J, Heid S, Klinke R. Congenital auditory deprivation reduces synaptic activity within the auditory cortex in a layer-specific manner. Cereb Cortex 2000;10:714–726
182. Kral A, Hartmann R, Tillein J, Heid S, Klinke R. Delayed maturation and sensitive periods in the auditory cortex. Audiol Neurootol 2001;6:346–362
183. Robertson D, Irvine DR. Plasticity of frequency organization in auditory cortex of guinea pigs with partial unilateral deafness. J Comp Neurol 1989;282:456–471
184. Rajan R, Irvine DR, Wise LZ, Heil P. Effect of unilateral partial cochlear lesions in adult cats on the representation of lesioned and unlesioned cochleas in primary auditory cortex. J Comp Neurol 1993;338:17–49
185. Reale RA, Brugge JF, Chan JC. Maps of auditory cortex in cats reared after unilateral cochlear ablation in the neonatal period. Brain Res 1987;431:281–290
186. Reser DH, Fishman YI, Arezzo JC, Steinschneider M. Binaural interactions in primary auditory cortex of the awake macaque. Cereb Cortex 2000;10:574–584
187. Kitzes LM. Some physiological consequences of neonatal cochlear destruction in the inferior colliculus of the gerbil, Meriones unguiculatus. Brain Res 1984;306:171–178
188. Kitzes LM, Hollrigel GS. Response properties of units in the posterior auditory field deprived of input from the ipsilateral primary auditory cortex. Hear Res 1996;100:120–130
189. Popelar J, Erre JP, Aran JM, Cazals Y. Plastic changes in ipsicontralateral differences of auditory cortex and inferior colliculus evoked potentials after injury to one ear in the adult guinea pig. Hear Res 1994;72:125–134
190. Bao S, Chang EF, Davis JD, Gobeske KT, Merzenich MM. Progressive degradation and subsequent refinement of acoustic representations in the adult auditory cortex. J Neurosci 2003;23:10765–10775
191. Polley DB, Heiser MA, Blake DT, Schreiner CE, Merzenich MM. Associative learning shapes the neural code for stimulus magnitude in primary auditory cortex. Proc Natl Acad Sci U S A 2004;101:16351–16356
192. Beitel RE, Schreiner CE, Cheung SW, Wang X, Merzenich MM. Reward-dependent plasticity in the primary auditory cortex of adult monkeys trained to discriminate temporally modulated signals. Proc Natl Acad Sci U S A 2003;100:11070–11075
193. Klinke R, Kral A, Heid S, Tillein J, Hartmann R. Recruitment of the auditory cortex in congenitally deaf cats by long-term cochlear electrostimulation. Science 1999;285:1729–1733
194. Giraud AL, Truy E, Frackowiak R. Imaging plasticity in cochlear implant patients. Audiol Neurootol 2001;6:381–393
195. Nishimura H, Hashikawa K, Doi K, et al. Sign language ‘heard’ in the auditory cortex. Nature 1999;397:116
196. Sadato N, Okada T, Honda M, et al. Cross-modal integration and plastic changes revealed by lip movement, random-dot motion and sign languages in the hearing and deaf. Cereb Cortex 2005;15:1113–1122
197. Petitto LA, Zatorre RJ, Gauna K, Nikelski EJ, Dostie D, Evans AC. Speech-like cerebral activity in profoundly deaf people processing signed languages: implications for the neural basis of human language. Proc Natl Acad Sci U S A 2000;97:13961–13966
198. Nishimura H, Doi K, Iwaki T, et al. Neural plasticity detected in short-and long-term cochlear implant users using PET. Neuroreport 2000;11:811–815
199. Hickok G, Bellugi U, Klima ES. The basis of the neural organization for language: evidence from sign language aphasia. Rev Neurosci 1997;8:205–222
200. Ponton CW, Eggermont JJ. Of kittens and kids: altered cortical maturation following profound deafness and cochlear implant use. Audiol Neurootol 2001;6:363–380
201. Eggermont JJ, Ponton CW. Auditory-evoked potential studies of cortical maturation in normal hearing and implanted children: correlations with changes in structure and speech perception. Acta Otolaryngol 2003;123:249–252
202. Sharma A, Dorman MF, Spahr AJ. A sensitive period for the development of the central auditory system in children with cochlear implants: implications for age of implantation. Ear Hear 2002;23:532–539
203. Ponton CW, Don M, Eggermont JJ, Waring MD, Kwong B, Masuda A. Auditory system plasticity in children after long periods of complete deafness. Neuroreport 1996;8:61–65
204. Vasama JP, Makela JP, Parkkonen L, Hari R. Auditory cortical responses in humans with congenital unilateral conductive hearing loss. Hear Res 1994;78:91–97
205. Vasama JP, Makela JP. Auditory pathway plasticity in adult humans after unilateral idiopathic sudden sensorineural hearing loss. Hear Res 1995;87:132–140
206. Vasama JP, Makela JP, Pyykko I, Hari R. Abrupt unilateral deafness modifies function of human auditory pathways. Neuroreport 1995;6:961–964
207. Vasama JP, Makela JP. Auditory cortical responses in humans with profound unilateral sensorineural hearing loss from early childhood. Hear Res 1997;104:183–190
208. Scheffler K, Bilecen D, Schmid N, Tschopp K, Seelig J. Auditory cortical responses in hearing subjects and unilateral deaf patients as detected by functional magnetic resonance imaging. Cereb Cortex 1998;8:156–163
209. Ponton CW, Vasama JP, Tremblay K, Khosla D, Kwong B, Don M. Plasticity in the adult human central auditory system: evidence from late-onset profound unilateral deafness. Hear Res 2001;154:32–44
210. Suzuki M, Kouzaki H, Nishida Y, Shiino A, Ito R, Kitano H. Cortical representation of hearing restoration in patients with sudden deafness. Neuroreport 2002;13:1829–1832
211. Khosla D, Ponton CW, Eggermont JJ, Kwong B, Don M, Vasama JP. Differential ear effects of profound unilateral deafness on the adult human central auditory system. J Assoc Res Otolaryngol 2003;4:235–249
212. Berthezene Y, Truy E, Morgon A, et al. Auditory cortex activation in deaf subjects during cochlear electrical stimulation. Evaluation by functional magnetic resonance imaging. Invest Radiol 1997;32:297–301
213. Herzog H, Lamprecht A, Kuhn A, Roden W, Vosteen KH, Feinendegen LE. Cortical activation in profoundly deaf patients during cochlear implant stimulation demonstrated by H2(15)O PET. J Comput Assist Tomogr 1991;15:369–375
214. Ito J, Sakakibara J, Iwasaki Y, Yonekura Y. Positron emission tomography of auditory sensation in deaf patients and patients with cochlear implants. Ann Otol Rhinol Laryngol 1993;102:797–801
215. Ito J. Auditory cortex activities in severely hearing-impaired and cochlear implant patients. Positron emission tomographic study. Adv Otorhinolaryngol 1993;48:29–34
216. Eggermont JJ, Ponton CW, Don M, Waring MD, Kwong B. Maturational delays in cortical evoked potentials in cochlear implant users. Acta Otolaryngol 1997;117:161–163
217. Lee DS, Lee JS, Oh SH, et al. Cross-modal plasticity and cochlear implants. Nature 2001;409:149–150
218. Naito Y, Hirano S, Honjo I, et al. Sound-induced activation of auditory cortices in cochlear implant users with post-and prelingual deafness demonstrated by positron emission tomography. Acta Otolaryngol 1997;117:490–496
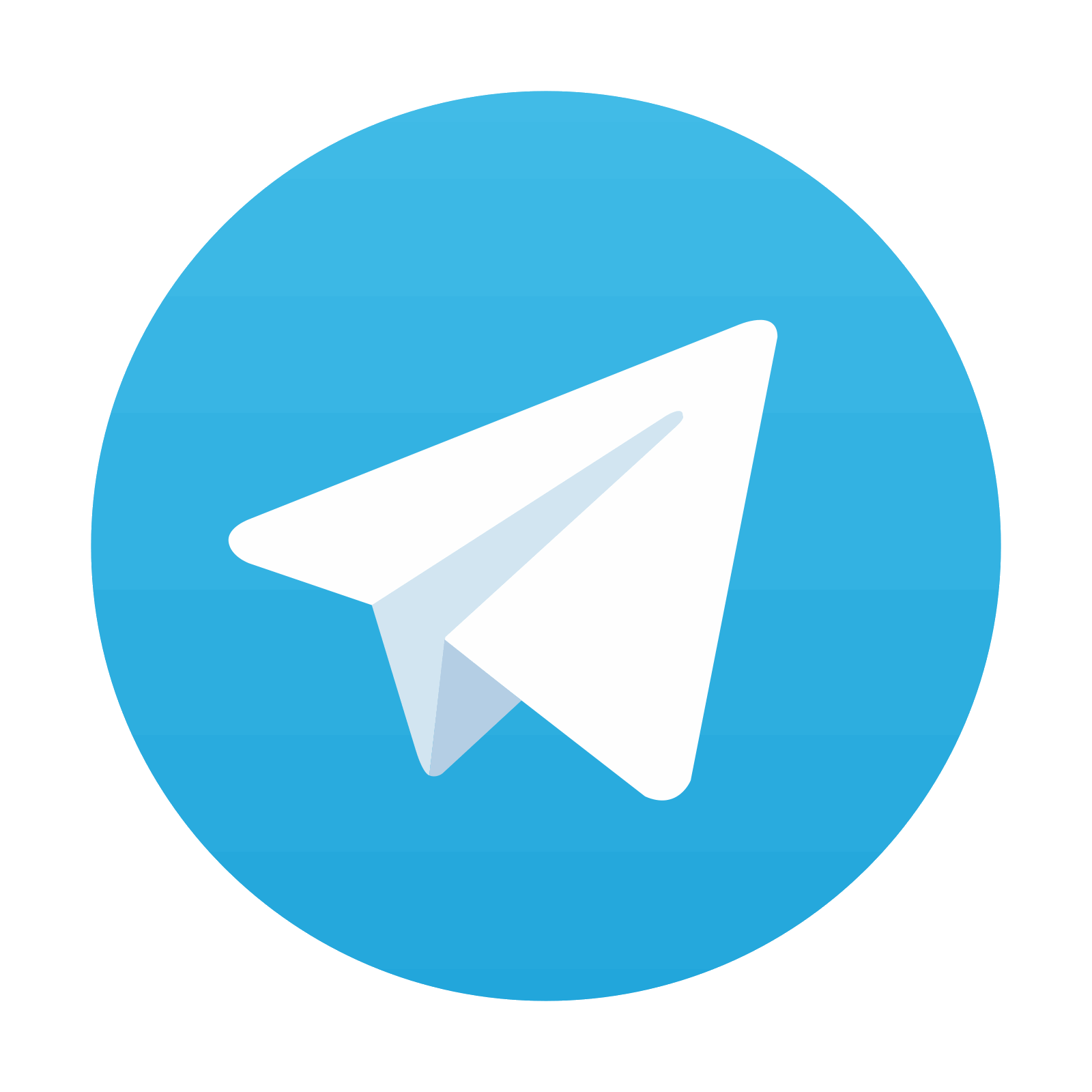
Stay updated, free articles. Join our Telegram channel
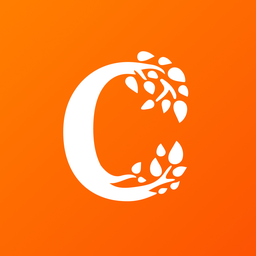
Full access? Get Clinical Tree
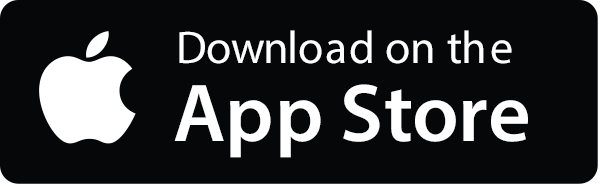
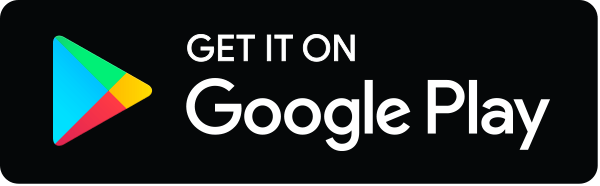