Clinical background
Key symptoms and signs
“Normal” color vision refers to the form of trichromatic color vision shared by most humans. It is mediated by three types of retinal cone photoreceptors, designated long- (L), middle- (M), and short- (S) wavelength cones. Each cone type is maximally sensitive to a different region of the visible spectrum ( Figure 62.1 ). The neural circuitry for color vision compares the outputs of the three cone types and generates neural signals that give rise to the percepts of six basic colors: blue, yellow, red, green, black, and white, and to their mixtures. Color vision defects can either be inherited as the consequence of gene defects that affect the function of one or more cone type, or they can be acquired secondary to disease or through exposure to neurotoxins. The hallmark feature of color vision defects is a reduction in the number of different colors that are seen as distinguishable from each other. The terms “protan” and “deutan” refer to color vision defects that result from abnormalities in the L and M cones and are collectively termed red–green color vision deficiency. These are characterized by diminished sensations of red and green, and this translates to a difficulty with several color combinations: (1) confusion between colors in the middle-to-long-wavelength region of the spectrum, which include green, yellow, orange, and red, particularly the pale versions of those colors and their very dark counterparts, olive, brown, and brick; (2) confusion between greenish and reddish colors, and gray, especially turquoise and gray and magenta or pink and gray; and (3) confusion between blue, violet, and purple. The term “tritan” refers to color vision deficits that result from abnormalities in the S cones and they are characterized by diminished sensations of blue and yellow difficulty, resulting in confusions between dark blue and black, yellow and yellow–green and white and also confusions among colors in the short-wavelength region of the spectrum: violet, purple, blue, and blue–green. Achromatopsia refers to complete or nearly complete absence of color perception (see glossary, below).

Quick reference for terminology for inherited color vision defects
- •
Protan: from the Greek for “the first”: defective color vision mediated by S cones and M cones with no L-cone function. A category of red–green color vision deficiency.
- •
Deutan: from the Greek for “the second”: color vision mediated by S cones and L cones with no M-cone function. A category of red–green color vision deficiency.
- •
Tritan: from the Greek for “the third”: color vision mediated by L and M cones with abnormal or no S-cone function. Blue–yellow color vision deficiency.
- •
Achromatopsia: vision mediated either by S cones and rods (blue-cone monochromacy) or rods only (rod monochromacy)
Glossary of official gene designations
- •
OPN1LW : X-chromosome gene encoding the opsin for the L-cone photopigment.
- •
OPN1MW : X-chromosome gene encoding the opsin for the M-cone photopigment.
- •
OPN1SW : chromosome 7 gene encoding the opsin for the S-cone photopigment.
- •
CNGA3 : gene encoding the alpha subunit of the cyclic nucleotide gated ion channel that photoreceptors use to modulate membrane potential in response to light.
- •
CNGB3 : gene encoding the beta subunit of the cyclic nucleotide gated ion channel that photoreceptors use to modulate membrane potential in response to light.
- •
GNAT2 : gene encoding the alpha subunit of transducin, the G-protein to which the cone photopigments, which are G-protein-coupled receptors, are coupled.
Historical development
The mechanisms of inherited color vision defects are far better understood than those of acquired defects, largely because inherited color vision deficiencies are extremely common and they are more tractable to investigation. The first recorded description of color vision deficiency was a report by John Dalton describing his own red–green defect, hence “daltonism” is the accepted term for inherited red–green color vision deficiency in many languages. Dalton hypothesized that his color vision defect was caused by blue tinted vitreous, which was disproven after his death in 1844, and in 1995, a molecular genetic study of Dalton’s preserved retinas allowed the basis of his defect to be identified: this was the deletion of the gene encoding the M-cone photopigment. Although the tendency for color vision defects to run in families and to skip generations has long been recognized, the underlying genetics has only been well characterized within the last 100 years. In the 1920s Waaler used results he collected from nearly 20 000 schoolchildren in Oslo to form the basis for his hypothesis that two separate genes on the X chromosome – one for L-cone sensitivity and another for M-cone sensitivity – accounted for the inheritance patterns of normal and defective color vision (2 1457), and over the next few decades his ideas were developed into a two-locus model of red–green color vision in which a series of alleles of the L-pigment gene were proposed to account for the range of protan red–green color vision defects and a separate set of alleles of the M-pigment gene accounted for the range of deutan red–green defects.
The next big breakthrough in understanding the biological basis of color vision and its defects came when Jeremy Nathans and colleagues cloned and sequenced the genes encoding the S-, M-, and L-cone opsins (see glossary above for definition), and demonstrated that the L- and M-opsin genes are, in fact, adjacent on the X chromosome. From his observations, Nathans hypothesized that color vision defects were not the result of independent mutations at separate L and M pigment gene loci, as had previously been believed, but were instead a consequence of genetic recombination between the L and M genes. Another outcome of the tandem arrangement of the photopigment genes as observed by Nathans is that expression of both the L and M genes is controlled by a by a shared DNA-regulatory element that, when deleted, causes blue-cone monochromacy. Finally, Nathans observed that there was variability in the number of X-linked opsin genes among people with normal color vision, and this presumably arose from unequal crossing over between X-chromosome pigment gene arrays.
Subsequently, point mutations in the S opsin gene were found to underlie inherited tritan defects. (Gunther 2006 #1115). The advent of methods for efficient genetic linkage analysis, which has been applied to families and populations affected by rod monochromacy, allowed the discovery of gene defects underlying these disorders.
Epidemiology
Inherited color vision defects
In the USA and western Europe, red–green color vision defects are extremely common, but the prevalence is about 19 times greater in males than in females because it is X-linked recessive. An estimated 1 in 12 males and 1 in 230 females is affected. The prevalence of red–green color vision defects varies with ethnicity. Caucasian populations in the USA and western Europe are reported to have the highest prevalence, with about 8% of males being affected. Native Fijians have the lowest, with fewer than 1% of males affected, and African and Japanese males have intermediate frequencies, with an estimated 4% of African and ∼2% of Japanese males being affected (reviewed in reference ). All other color vision defects are relatively rare.
Inherited blue–yellow color vision defects affect males and females equally. Prevalence values reported in the literature range from 1 in 500 to 1 in 65 000. Estimating the prevalence has been challenging because most commonly used clinical color vision tests do not adequately test for color discrimination in the blue–yellow region of the spectrum. Achromotopsias are also extremely rare, and include both blue-cone monochromacy and rod monochromacy. The prevalence of blue-cone monochromacy, which is an X-linked trait and therefore preferentially affects males, has been estimated to be about 1 in 100 000, whereas rod monochromacy affects males and females equally, with prevalence estimates ranging from 1 in 20 000 to 1 in 50 000.
Genetics
Inherited color vision defects
The vast majority of inherited color vision defects are caused by mutations that affect the expression or function of the cone photopigments. Photopigments are comprised of a protein, termed opsin, attached to an 11- cis retinal chromophore. The cone photopigments all use the same chromophore, but different opsins. Three opsins corresponding to each cone type, L, M, and S, are encoded by three genes designated OPN1LW , OPN1MW , and OPN1SW , respectively. OPN1LW and OPN1MW are located on the X chromosome at Xq28, and OPN1SW resides on chromosome 7 at 7q32.
Blue–yellow color vision deficiency is inherited as an autosomal-dominant trait caused by mutations in the OPN1SW gene. Both the common red–green color vision defects and the relatively uncommon blue-cone monochromacy are X-linked, being caused by mutations and gene rearrangements of the X-chromosome opsin genes, OPN1LW or OPN1MW . For red–green defects the gene rearrangements result in the absence of one cone type, L or M, but for blue-cone monochromacy the mutations result in the absence of both L and M cones. Rod monochromacies are autosomal recessive and causative mutations have been identified in the three genes: the CNGA3 and CNGB3 genes encoding the α and β subunits, respectively, of the cyclic nucleotide gated ion channel that is essential for cone photoreceptors to modulate membrane potential in response to light absorption, and in the GNAT2 gene that encodes the α subunit of cone transducin. These gene defects result in the loss of function for all three cone types.
Diagnostic workup
There are a large number of different tests used in the diagnosis of color vision defects. Pseudoisochromatic plates are the most familiar color vision tests. The most widely used examples are Ishihara’s test of color vision and the Hardy, Rand and Rittler (HRR) pseudoisochromatic plates. These tests each consist of a book containing a series of printed colored plates. Each plate is designed to conceal a hidden figure that can be seen by a person with normal color vision but is obscured for people with particular color vision deficiencies.
Arrangement tests – the best examples are the Farnsworth–Munsell 100 hue test and its abridged version, the Farnsworth–Munsell dichotomous D-15 test – consist of a series of colored disks in which the color changes from one to the next in small steps. The caps are mixed up and the task is to “arrange” the disks “in order” so that each disk is next to the color closest to it in appearance. Color vision defects are diagnosed by the misordering of the disks.
The anomaloscope is an instrument that contains an optical system that produces two side-by-side lighted fields, one monochromatic amber and the other a mixture of red and green light. The subject adjusts the ratio of red-to-green light in the mixture until it exactly matches the amber monochromatic light. People who have inherited red–green color anomalies in which either the L or M pigment is shifted in spectral peak compared to normal will require either a higher or lower ratio of red-to-green light in the mixture. This test is extraordinarily sensitive to genetic alterations in the spectral sensitivities of the photopigments and will detect them even in individuals in whom the alteration in the photopigment has little or no effect on the person’s ability to discriminate between different colors. Because of its extreme sensitivity in detecting the presence of anomalous photopigments, the anomaloscope is often referred to as the “gold standard” for diagnosing inherited red–green color vision deficiencies. However, while an abnormal result on this test does indicate a photopigment abnormality, some mild photopigment abnormalities are associated with little or no loss in color discrimination ability.
Differential diagnosis
Acquired color vision defects are usually not present from birth, are often accompanied by other visual problems including decreased acuity and visual field defects, can affect one or both eyes, and usually become progressively worse. Common causes are optic neuropathies (e.g., toxic, inflammatory, nutritional) or retinopathies (e.g., toxic, paraneoplastic, degenerative). These are discussed at length in other chapters. Inherited color vision deficiencies are suggested by congenital onset, stability, and a compatible family history ( Box 62.1 ). They are most reliably distinguished from acquired deficiencies by genetic testing, which is not commercially available at present.
Inherited color vision defects
Red–green color vision defects
- •
X-linked
- •
Very common
- •
More common in males than females
- •
Most often caused by deletions and rearrangements of the genes encoding the long- and middle-wavelength-sensitive cone opsins on the X chromosome
Blue–yellow color vision defects
- •
Autosomal dominant
- •
Relatively rare
- •
Caused by mutations in gene encoding short-wavelength cone opsin that produces amino acid substitutions
Acquired color vision defects
- •
Caused by diseases that affect nerve function or by exposure to neurotoxins
- •
Because red–green color vision defects are so common, it is not unusual for a person with an acquired color vision defect also to have an inherited color vision defect, making diagnosis of the acquired defect more challenging
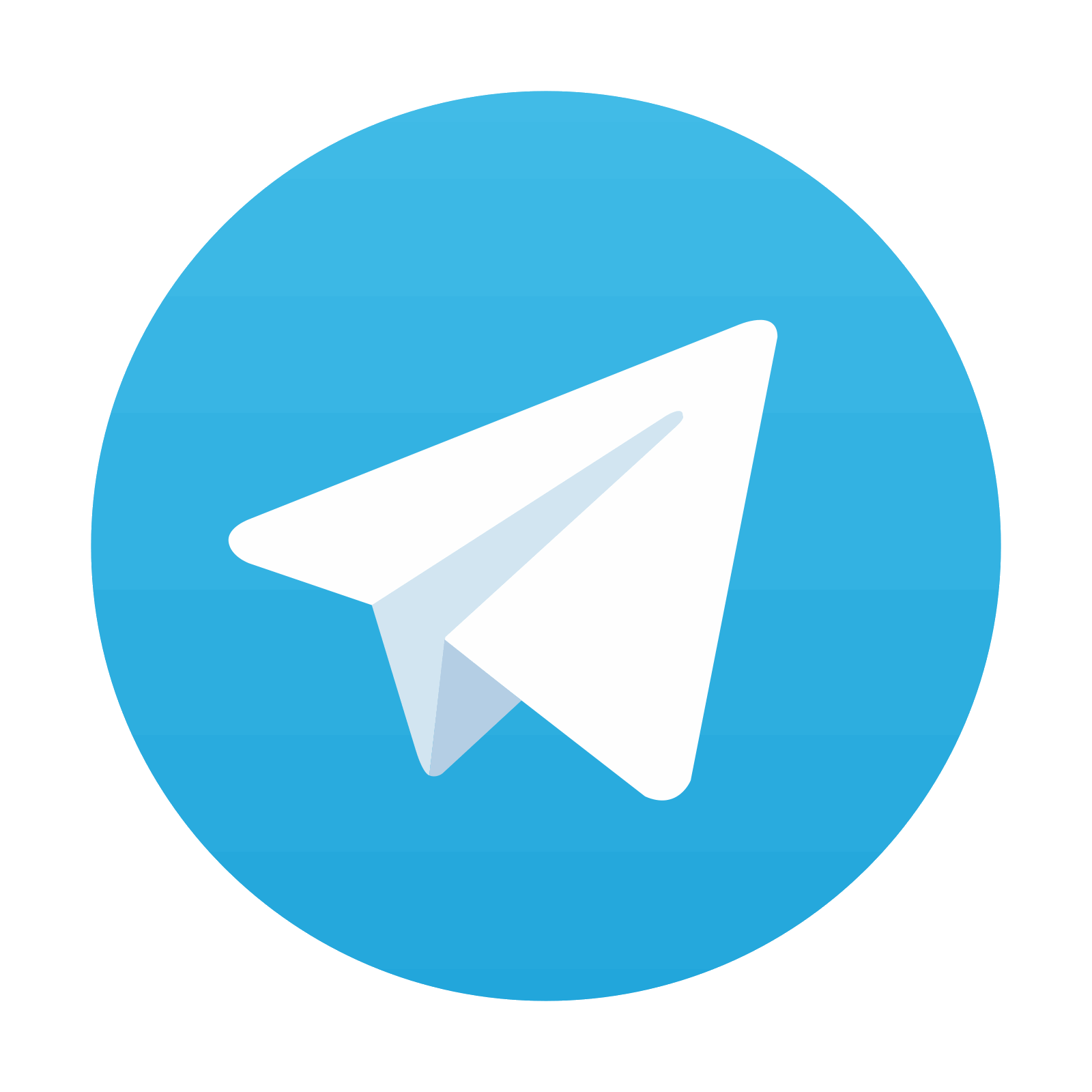
Stay updated, free articles. Join our Telegram channel
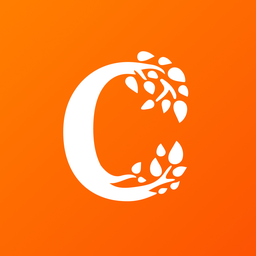
Full access? Get Clinical Tree
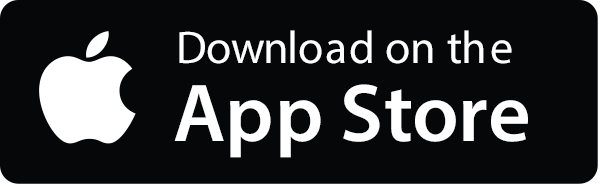
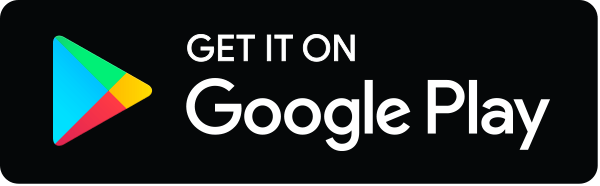
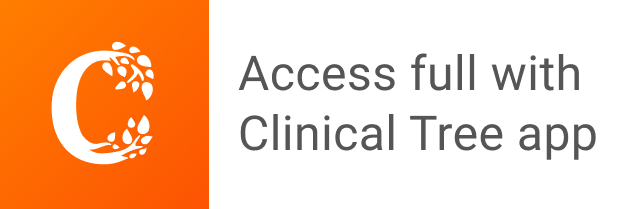