Optic Nerve, Retina, and Choroid
Glaucoma is characterized by progressive atrophy of the optic nerve head secondary to the loss of optic nerve fiber. Because it is this pathologic alteration that leads to the irreversible loss of vision, an understanding of glaucomatous optic atrophy is essential in the diagnosis and management of glaucoma.
ANATOMY AND HISTOLOGY
Terminology
In the context of a discussion on glaucoma, the optic nerve head is defined as the distal portion of the optic nerve that is directly susceptible to intraocular pressure (IOP) elevation. In this sense, the optic nerve head extends anteriorly from the retinal surface to the myelinated portion of the optic nerve that begins just behind the sclera, posterior to the lamina cribrosa. The term optic nerve head is generally preferred over optic disc because the latter suggests a flat structure without depth. However, the terms disc and papilla are frequently used when referring to the portion of the optic nerve head that is clinically visible by ophthalmoscopy (1). It is the optic nerve head and nerve fiber layer containing retinal ganglion cell (RGC) axons that are most clearly associated with glaucomatous vision loss (Fig. 4.1).
Figure 4.1 A: Optic nerve head with physiologic enlarged cupping demonstrating robust, symmetric, and healthy RNFL. B: Glaucomatous optic nerve showing an inferotemporal notch and corresponding loss of the RNFL that is appreciated by “baring” of the retinal vessels. The point of the (arrows) delimits the RNFL defect.
General Description
The optic nerve head comprises the nerve fibers that originate in the ganglion cell layer of the retina and converge upon the nerve head from all points in the fundus. At the surface of the nerve head, these RGC axons bend acutely to exit the globe through a fenestrated scleral canal, called the lamina cribrosa. In the nerve head, the axons are grouped into approximately 1000 fascicles, or bundles, and are supported by astrocytes. There is considerable variation in the size of the optic nerve head. In one study, the diameter varied from 1.18 to 1.75 mm (2). Other studies have revealed ranges of 0.85 to 2.43 mm in the shortest diameter and 1.21 to 2.86 mm in the longest (3), or a mean of 1.88 mm vertically and 1.77 mm horizontally (4). The disc area may range from 0.68 mm2 to 4.42 mm2 (3). In a large, population-based study, the average disc area was 2.42 mm2 (5). In a different study, the average disc area was 2.56 mm2 when measured by the Heidelberg retina tomograph (HRT) and 2.79 mm2 by the analysis of disc photographs (6). When optic nerve head area and neuroretinal rim area were determined in 36 radial 10-degree segments on stereophotographs, cup area had stronger correlation with the disc area than the rim area, suggesting that correction for disc size may be more important for cup area than for rim area (7). Another study showed a positive correlation between the optic disc size and the thickness of the peripapillary retinal nerve fiber layer (RNFL) (8).
Studies using a confocal scanning laser tomograph showed that in healthy eyes the neuroretinal rim area and optic disc diameter have a higher correlation with the optic nerve head configuration than with age, sex, or refractive error (9). The diameter and the area may vary depending on the definition of the edge of the optic disc and methods of measurement (4,10,11). Therefore, some authors have suggested applying various formulas to correct magnification of images when comparing disc measurements on different instruments (12,13).
The diameter of the nerve expands to approximately 3 mm just behind the sclera, where the neurons acquire a myelin sheath. The optic nerve head is also the site of entry and exit of the retinal vessels. This vascular system supplies some branches to the optic nerve head, although the predominant blood supply for the nerve head comes from the ciliary circulation.
Divisions of the Optic Nerve Head
The nerve head may be arbitrarily divided into four portions from anterior to posterior (14) (Fig. 4.2).
Figure 4.2 Divisions of the optic nerve head. A: Surface nerve fiber layer. B: Prelaminar region. C: Lamina cribrosa region. D: Retrolaminar region.
Surface Nerve Fiber Layer
The innermost portion of the optic nerve head is composed predominantly of nerve fibers. In the rhesus monkey, this layer is 94% RGC axons and 5% astrocytes (15). The axonal bundles acquire progressively more interaxonal glial tissue in the intraocular portion of the nerve head as this structure is followed posteriorly (15).
Prelaminar Region
The prelaminar region is also called the anterior portion of the lamina cribrosa (16). The predominant structures at this level are nerve axons and astrocytes, with a significant increase in the quantity of astroglial tissue.
Lamina Cribrosa Region
This portion contains fenestrated sheets of scleral connective tissue and occasional elastic fibers. Astrocytes separate the sheets and line the fenestrae (16), and the fascicles of neurons leave the eye through these openings.
Retrolaminar Region
This area is characterized by a decrease in astrocytes and the acquisition of myelin that is supplied by oligodendrocytes. The axonal bundles are surrounded by connective tissue septa.
The posterior extent of the retrolaminar region is not clearly defined. An India ink study of monkey eyes showed nonfilling with the ink for 3 to 4 mm behind the lamina cribrosa when the IOP was elevated (17). However, a similar study using unlabeled microspheres showed an increased blood flow in the retrolaminar region close to the lamina even when the IOP was elevated high enough to stop retinal blood flow (18).
Vasculature
Arterial Supply
Posterior ciliary artery circulation is the main source of blood supply to the optic nerve head (19), except for the nerve fiber layer—which is supplied by the retinal circulation. The blood supply in the optic nerve head has a sectoral distribution (20). The four divisions of the optic nerve head correlate roughly with a four-part vascular supply (Fig. 4.3).
Figure 4.3 Vascular supply of the optic nerve head. CRA, central retinal artery;RPC, radial peripapillary capillaries; PV, pial vessels; SPCA, short posterior ciliary arteries; PCV, peripapillary choroidal vessels; ZH, “circle” of Zinn–Haller.
The surface nerve fiber layer is mainly supplied by arteriolar branches of the central retinal artery, which anastomose with vessels of the prelaminar region and are continuous with the peripapillary retinal and long radial peripapillary capillaries (14,19,21). The temporal region may also be supplied by one or more of the ciliary-derived vessels from the posterior ciliary artery circulation in the deeper prelaminar region, which may occasionally enlarge to form cilioretinal arteries (14). The cilioretinal artery, when present, usually supplies the corresponding sector of the surface layer (20). In elderly rhesus monkeys, central retinal artery occlusion for less than 100 minutes produced no apparent evidence of optic nerve damage. However, longer occlusion produced a variable degree of damage (22).
The prelaminar and laminar regions are supplied primarily by short posterior ciliary arteries, which form a perineural, circular arterial anastomosis at the scleral level, called the circle of Zinn–Haller (14,19,21,23). Branches from this circle penetrate the optic nerve to supply the prelaminar and laminar regions and the peripapillary choroid (19). The circle is not present in all eyes, in which case direct branches from the short posterior ciliary arteries supply the anterior optic nerve. The peripapillary choroid may also minimally contribute to anterior optic nerve (14,19,21,23).
The retrolaminar region is supplied by both the ciliary and retinal circulations, with the former coming from recurrent pial vessels. Medial and lateral perioptic nerve short posterior ciliary arteries anastomose to form an elliptical arterial circle around the optic nerve, which has also been referred to as the circle of Zinn–Haller (24,25). This perioptic nerve arteriolar anastomosis, which supplies the retrolaminar optic nerve, was found to be complete in 75% of 18 human eyes in one study (24). The central retinal artery provides centripetal branches from the pial system and frequently, but not always, gives off centrifugal vessels (20).
Continuity between small vessels from the retrolaminar region to the retinal surface has been observed (21), and the optic nerve head microvasculature is said to represent an integral part of the retina–optic nerve vascular system (23).
Capillaries
Although derived from both the retinal and ciliary circulations, the capillaries of the optic nerve head resemble more closely the features of retinal capillaries than of the choriocapillaris. These characteristics include (a) tight junctions, (b) abundant pericytes, and (c) nonfenestrated endothelium (23). They do not leak fluorescein and may represent a nerve–blood barrier, supporting the concept of the retina–nerve vasculature as a continuous system with the central nervous system (21,23). The capillaries decrease in number posterior to the lamina, especially along the margins of the larger vessels (26).
Venous Drainage
The venous drainage from the optic nerve head is almost entirely through the central retinal vein (19), although a small portion may occur through the choroidal system (27). Occasionally, these communications are enlarged as retinociliary veins, which drain from the retina to the choroidal circulation, or cilio-optic veins, which drain from the choroid to the central retinal vein (28).
Astroglial Support
Astrocytes provide a continuous layer between the nerve fibers and blood vessels in the optic nerve head (29). In the rhesus monkey, astrocytes occupy 5% of the nerve fiber layer, increase to 23% of the laminar region, and then decrease to 11% in the retrolaminar area (15). The astrocytes are joined by “gap junctions,” which resemble tight junctions but have minute gaps between the outer membrane leaflets (30).
Thick- and thin-bodied astrocytes have been described. The thin-bodied astrocytes accompany the axons in the nerve fiber layer, and the thick-bodied astrocytes direct axons in the prelaminar region toward the laminar region (31).
The astroglial tissue also provides a covering for portions of the optic nerve head (Fig. 4.4). The internal limiting membrane of Elschnig separates the nerve head from the vitreous and is continuous with the internal limiting membrane of the retina (29,32–34). The central portion of the internal limiting membrane is referred to as the central meniscus of Kuhnt (33). Although the central meniscus of Kuhnt is traditionally described as a central thickening of the internal limiting membrane, ultrastructural studies of the monkey optic nerve head revealed a thinning of 20 nm centrally, which thickened to 70 nm peripherally (34). The Müller cells are a major constitutional element of the intermediary tissue of Kuhnt (35), which separates the nerve from the retina, whereas the border tissue of Jacoby separates the nerve from the choroid (16,33).
Figure 4.4 Supportive structures of the optic nerve head: internal limiting membrane of Elschnig (a); continuous with the internal limiting membrane of the retina (b); central meniscus of Kuhnt (c); intermediary tissue of Kuhnt (d); border tissue of Jacoby (e); border tissue of Elschnig (f ); lamina cribrosa (g); meningeal sheaths (h).
Astrocytes also play a major role in the remodeling of the extracellular matrix of the optic nerve head and synthesizing growth factors and other cellular mediators that may affect the axons of the RGCs and contribute to health or susceptibility to disease (36).
Connective Tissue Support
Lamina Cribrosa
This structure is not simply a porous region of the sclera but also a specialized extracellular matrix that consists of fenestrated sheets of connective tissue and occasional elastic fibers lined by astrocytes (16,37). Astrocytes may respond to changes in IOP in glaucoma, leading to axonal loss and RGC degeneration at the level of lamina cribrosa (36). Extracellular matrix components in the lamina cribrosa differ from those in sclera or pial septa (38), which may be important in the pathogenesis of glaucomatous optic nerve damage. Hyaluronate was found surrounding the myelin sheaths in the retrolaminar nerve, playing an important role in the maintenance of the hydrodynamic properties of the extracellular matrix. Hyaluronate decreases with age and is further reduced in eyes with chronic open-angle glaucoma (COAG), possibly increasing susceptibility to elevated IOP (39). The lamina cribrosa has also been found to be significantly thinner in glaucomatous eyes than in nonglaucomatous eyes (40).
Analysis of the pores in the lamina cribrosa with a confocal scanning laser ophthalmoscope shows nearly round pores in the eyes with physiologic cupping, whereas eyes with COAG frequently have compressed pores (41). There are regional differences in the fenestration or pores through which the axons pass. The superior and inferior portions, compared with the nasal and temporal regions, have larger single pore areas and summed pore areas and thinner connective tissue and glial cell support (42–45) (Fig. 4.5). The ratio of single and summed pore areas between the laminar regions decreases with increasing lamina cribrosa area, but does not correlate with age or sex (45). A majority of RGC axons take a direct course through the lamina cribrosa (46), but about 10% of axons exit more peripherally, where the lamina cribrosa is more curvilinear, which may influence the regional susceptibility for glaucomatous optic nerve fiber loss (47). The size of the laminar openings for the retinal vessels does not correlate with the lamina cribrosa area (45).
Figure 4.5 Gross anatomic photograph of lamina cribrosa showing central openings for central retinal vessels (arrow) and surrounding fenestrae of lamina for passage of axon bundles. Note larger size of fenestrae in superior and inferior quadrants. S, superior; T, temporal. (Courtesy of Harry A. Quigley, MD.)
As mentioned previously, the lamina cribrosa of the human optic nerve head contains a specialized extracellular matrix composed of collagen types I through VI, laminin, and fibronectin (48–50). Studies of young human donor eyes show that the cribriform plates are composed of a core of elastin fibers with a sparse, patchy distribution of collagen type III, coated with collagen type IV and laminin (48). Cell cultures of human lamina cribrosa reveal two cell types, which appear to synthesize this extracellular matrix (51). The expression of mRNA for collagen types I and IV in both fetal and adult human optic nerve heads suggests that these extracellular matrix proteins are synthesized in this tissue throughout life (52). Proteoglycans, which are macromolecular components of connective tissue believed to have a role in the organization of other extracellular matrix components and in the hydration and rigidity of tissue, have been identified in the cores of the laminar plates in association with collagen fibers (53,54). Cell adhesive proteins, including vitronectin and thrombospondin, have been found in human lamina cribrosa (38). Abnormalities of this extracellular matrix in the lamina cribrosa may influence optic nerve function and its susceptibility to glaucomatous damage caused by elevated IOP. Lamina cribrosa cells from glaucomatous eyes express more profibrotic genes than cells from normal lamina cribrosa do (55). These differences in extracellular matrix probably translate into difference in biomechanical properties (56,57).
Nerve Sheaths
A rim of connective tissue, the border tissue of Elschnig, occasionally extends between the choroid and optic nerve tissues, especially temporally (33) (Fig. 4.4). Posterior to the globe, the optic nerve is surrounded by meningeal sheaths (pia, arachnoid, and dura), which consist of connective tissue lined by meningothelial cells, or mesothelium (58). Lymphatic capillaries in the dura of the human optic nerve have been described (59). Vascularized connective tissue extends from the undersurface of the pia mater to form longitudinal septa, which partially separate the axonal bundles in the intraorbital portion of the optic nerve (33).
Axons
Retinal Nerve Fiber Layer
As the axons traverse the nerve fiber layer from the ganglion cell bodies to the optic nerve head, they are distributed in a characteristic pattern (Fig. 4.6). Fibers from the temporal periphery originate on either side of a horizontal dividing line, the median raphe, and arch above or below the fovea as the arcuate nerve fibers, while those from the central retina, the papillomacular fibers, and the nasal fibers take a more direct path to the nerve head. The significance of this anatomy to the visual field defects of glaucoma is discussed in Chapter 5. The axons in monkeys and rabbits are grouped into fiber bundles by tissue tunnels composed of elongated processes of Müller cells (60–62). These bundles, especially on the temporal side, become larger as they approach the nerve head, primarily because of lateral fusion of bundles (63), and are normally visible by ophthalmoscopy as retinal striations (62). The axons in the bundles vary in size, with larger fibers coming from the more peripheral retina (63). One study also demonstrated that intra-RGC axons contain numerous bulb-shaped varicosities in humans of different ages (64).
Figure 4.6 Distribution of retinal nerve fibers. Note arching above and below the fovea of fibers temporal to the optic nerve head. Inset depicts crosssectional arrangement of axons, with fibers originating from peripheral retina running closer to choroid and periphery of optic nerve, while fibers originating nearer to the nerve head are situated closer to the vitreous and occupy a more central portion of the nerve.
Axons in Optic Nerve Head
The arcuate nerve fibers occupy the superior and inferior temporal portions of the optic nerve head, with axons from the peripheral retina taking a more peripheral position in the nerve head (Fig. 4.6) (65). The arcuate fibers are the most susceptible to early glaucomatous damage. The papillomacular fibers spread over approximately one third of the distal optic nerve, primarily inferior temporally, where the axonal density is higher (66,67). They intermingle with extramacular fibers, which may explain the retention of central vision in early glaucomatous optic atrophy.
The mean axonal population in the normal human optic nerve head, as measured by computed image analysis of sections throughout the nerve, ranges from approximately 700,000 fibers to 1.2 million fibers (67–70). The optic nerve fiber count has been shown to increase significantly with the optic nerve head area in human and monkey eyes, although another study of human eyes showed no such correlation (69,71,72). A positive correlation has also been demonstrated between the retinal photoreceptor count and optic nerve area (73). The reported mean axonal fiber diameter ranges from 0.65 to 1.10 µm (67,68,74). Axons of all sizes are mixed throughout the nerve area, although higher mean diameters appear to be more common in the nasal segment (67).
EMBRYOLOGY OF THE RETINA AND OPTIC NERVE
The retina and optic nerve develop from the optic cup and the contiguous optic stalk (75–80).
The inner layer of the cup contains the pluripotent retinal progenitor cells, which differentiate in a specific chronologic sequence and defined histogenic order into the final seven retinal cell types (see Fig. 1.3 in Chapter 1). In general, the RGCs differentiate first (81,82), followed by the cone photoreceptors, amacrine cells, horizontal cells, and finally, the rod photoreceptors, bipolar cells, and Müller cells. Retinal neurogenesis starts in the central optic cup region and then fans out concentrically in a wavelike pattern into the periphery. There is a basic topographic organization of the optic cup with dorsoventral and nasotemporal patterning (83), which involves certain genetic cues, including that of the Otx genes (84).
The optic fissure of the optic stalk closes to convert it into a cylinder, into which the RGC axons grow. The lumen of the optic stalk is obliterated by axons by approximately the third fetal month. Apoptosis, or selective cell death, and cell cycle regulators are important in normal ocular development (85–87). The optic nerve axon count in humans peaks at approximately 3.7 million by fetal week 16 to 17 and then rapidly declines to near adult levels of around 1 million by term (88). Epithelial cells in the walls of the stalk differentiate into the neuroglia of the optic nerve. Mesenchymal tissue gives rise to the optic nerve septa in the third month and to the lamina cribrosa in the final month of gestation.
Key regulatory genes involved in the early development of the eye and the fate of retinal cells include Pax6, Rx1, Six3/6, Lhx2, and certain basic helix–loop–helix transcription factors. The expression of these genes and their effect on retinal neurogenesis and differentiation are considered “cell-intrinsic” mechanisms, whereas “extrinsic” mechanisms include thyroid hormones and their receptors, fibroblast growth factors and other “growth factors,” hedgehog proteins, various neurotrophins, and nitric oxide (75,89–95).
The optic nerve cross-sectional area reaches 50% of the adult size by 20 weeks’ gestation, 75% at birth, and 95% before 1 year of age (96). At birth, the optic nerve is nearly unmyelinated (97), and myelination, which proceeds from the brain to the eye during gestation, is largely completed in the retrolaminar region of the optic nerve by the first year of life (98). The connective tissue of the lamina cribrosa is also incompletely developed at birth, which may account for the increased susceptibility of the infant nerve head to glaucomatous cupping and its potential for reversible cupping (99). With increasing age, the cores of the cribriform plates enlarge, and the apparent density of collagen types I, III, and IV and elastin increases (100,101). Not only does elastin increase with age, but also elastic fibers become thicker, tubular, and surrounded by densely packed collagen fibers (101). Proteoglycan filaments in the human lamina cribrosa also decrease in length and diameter with age (102). Also with increasing age, there appears to be a progressive loss of axons with a decrease of the nerve fiber layer thickness (103,104) and a corresponding increase in the cross-sectional area occupied by the leptomeninges and fibrous septa (67–70). The loss of axons has been estimated to be between 4000 and 12,000 per year, with most studies nearer the lower figure (67,69,70,105). One study suggested a selective loss of large nerve fibers with age (68), although this has not been confirmed by others (67,74).
PATHOPHYSIOLOGY OF GLAUCOMATOUS OPTIC NERVE DAMAGE
Theories
The pathogenesis of glaucomatous optic atrophy has remained a matter of controversy since the mid-19th century, when two concepts were introduced in the same year. In 1858, Müller (106) proposed that the elevated IOP led to direct compression and death of the neurons (the mechanical theory), while von Jaeger (107) suggested that a vascular abnormality was the underlying cause of the optic atrophy (the vascular theory). In 1892, Schnabel (108) proposed another concept in the pathogenesis of glaucomatous optic atrophy, suggesting that atrophy of neural elements created empty spaces, which pulled the nerve head posteriorly (Schnabel cavernous atrophy).
Initially, the mechanical theory received the greatest support (109–111). This concept held sway through the first quarter of the 20th century until LaGrange and Beauvieux (112) popularized the vascular theory in 1925. In general, this belief held that glaucomatous optic atrophy was secondary to ischemia, whether the primary result of the elevated IOP or an unrelated vascular lesion (113–115). In 1968, however, the role of axoplasmic flow in glaucomatous optic atrophy was introduced (116), which revived support for the mechanical theory, but did not exclude the possible influence of ischemia.
Evidence
Continued investigation into the pathogenesis of glaucomatous optic atrophy has led to the following bodies of information.
Anatomic and Histopathologic Studies
Histopathologic observations of human eyes with glaucoma provide the most direct method of studying the alterations associated with glaucomatous optic atrophy, although they do not fully explain the mechanisms that caused the damage. One of the limiting factors has been that many of the specimens studied have come from eyes with advanced glaucomatous change, which led to possible misconceptions regarding the early pathogenic features. More recent studies, which have attempted to correlate clinical observations with histopathologic changes in optic nerve heads from eyes with varying stages of glaucoma, appear to clarify many of these points.
Glial Alterations
It was once suggested that loss of astroglial supportive tissue precedes neuronal loss (117), which was thought to explain the early and reversible cupping in infants (118). However, subsequent studies have shown that glial cells are not selectively lost in early glaucoma and are actually the only remaining cells after loss of axons in advanced cases (119,120).
Vascular Alterations
It was also once proposed that loss of small vessels in the optic nerve head accompanies atrophy of axons (121), and one histologic study suggested a selective loss of retinal radial peripapillary capillaries in eyes with chronic glaucoma (122). However, subsequent investigations revealed neither a correlation between atrophy of this vascular system and visual field loss nor a major selective loss of optic nerve head capillaries in human eyes with glaucoma (119,120,123,124). In animal models of optic atrophy, created by either sustained IOP elevation, sectioning of the optic nerve, or photocoagulation of the RNFL, the resulting disc pallor was not associated with a decrease in the ratio of capillaries to neural tissue, although the caliber of the vessels diminished (124–128). Instead, these studies showed a proliferation or reorganization of glial tissue, which obscures ophthalmoscopic visualization of the vessels (125,126,128).
Alterations of the Lamina Cribrosa
Backward bowing of the lamina cribrosa has long been recognized as a characteristic feature of late glaucomatous optic atrophy (129,130), and as an early change in the infant eye with glaucoma (99). Further study, however, has suggested that alterations in the lamina may actually be a primary event in the pathogenesis of glaucomatous optic atrophy. In enucleated human eyes, acute IOP elevation causes a backward bowing of the lamina (131,132), and similar changes are observed in primate glaucoma models (133,134) with compensatory remodeling and fibrosis (135).
Most of the posterior displacement occurred in the peripheral lamina cribrosa, corresponding to the region of early axonal loss (132). In a histopathologic evaluation of 25 glaucomatous human eyes, compression of successive lamina cribrosa sheets was the earliest detected abnormality, and backward bowing of the entire lamina occurred later and involved primarily the upper and lower poles (136).
In the early stages of adult glaucoma, the magnitude of backward bowing is not sufficient to explain the ophthalmoscopically observed cupping, but may be enough to produce a pressure gradient along the axoplasm of exiting optic nerve axons, compromise the circulation (137), and cause compression of the axons. It has been suggested that the structure of the lamina cribrosa may be an important determinant in the susceptibility of the optic nerve head to damage from elevated IOP (119,120). However, racial comparison of the relative connective tissue support and regional pore size of the lamina cribrosa did not explain the increased susceptibility of blacks to glaucomatous damage (138). The extracellular matrix of the lamina cribrosa may play an important role in the progression of glaucomatous damage (139–141). In glaucomatous monkey eyes, increased collagen type IV and laminin lined the margins of the laminar beams (140,141), and collagen types I, III, and IV were found in the pores of the beams (140). Elastin, which is the major protein of elastic fibers and responsible for elastic recoil, appeared curled instead of straight and seemed disconnected from other elements of the connective tissue matrix in glaucomatous eyes of humans and monkeys (142). Elastin mRNA expression in human eyes with COAG suggests synthesis of abnormal elastic fibers (143). These changes may be secondary to long-standing elevation of IOP and may modify the course of glaucomatous optic atrophy.
Axonal Alterations
The actual cause of early optic nerve head cupping in glaucoma appears to be the loss of axonal tissue (119,120,144). Experimental models of primate eyes exposed to chronic IOP elevation suggest that the damage is associated with a posterior and lateral displacement of the lamina cribrosa, which compresses the axons and disrupts axoplasmic flow (145). The damage first involves axonal bundles throughout the nerve with somewhat greater involvement of the inferior and superior poles (136). With continued optic nerve damage, the susceptibility of the polar zones becomes more prominent (Fig. 4.7) (119,120,136,144). Histologic studies of both monkey and human optic nerves indicate that nerve fibers larger than the normal mean diameter atrophy more rapidly in glaucomatous eyes, although no fiber size is spared from damage (146,147). This preferential loss of large fibers appears to be due to a higher proportion of the fibers in the inferior and superior poles, and an inherent susceptibility to injury by glaucoma (146,147).
Figure 4.7 A: Light microscopic view of normal optic nerve head on cross section with darkly staining axon bundles and intervening glial supportive tissue surrounding openings for central retinal vessels. B: Light microscopic cross-sectional view of optic nerve head with glaucomatous atrophy showing loss of axon bundles predominantly in the inferior and superior quadrants (compare with normal nerve head in A). INF, inferior; NAS, nasal; SUP, superior; TEM, temporal. (Courtesy of Harry A. Quigley, MD.)
In the retina of glaucomatous monkey eyes, there is also a selective loss of the larger ganglion cells in both the midperiphery and fovea, and it has been suggested that psychophysical testing should be aimed at these cells in the early stages of glaucoma (148,149). The same animal studies suggest that RGCs in glaucoma die by apoptosis, a genetically programmed process of cell death, characterized histologically by chromatin condensation and intracellular fragmentation (150). This apoptosis is possibly related to loss of trophic influences resulting from inhibited transmission of neurotrophic signals from axon terminals to neuronal cell bodies; histologic studies have also shown a significant decrease of corpora amylacea, which are homogeneous oval bodies believed to correlate with axonal degeneration, in RGCs and the optic nerve of human eyes with advancing stages of glaucoma (151,152). One study revealed a significant reduction in photoreceptor count in human eyes with angle-closure glaucoma associated with trauma (153), although this was not observed in human eyes with COAG or in monkey eyes with experimental glaucoma (154,155).
Secondary degeneration has been reported to occur after experimental injury of RGCs, causing loss of neighboring RGCs as an indirect effect of the injury and death of transected RGCs. Glutamate levels in the vitreous did not increase at 3 months after injury, suggesting the need for further investigations of the mechanisms of secondary degeneration (156).
Blood-Flow Studies
Blood flow in the optic nerve head of cats is relatively high compared with that in more posterior portions of the nerve, and autoregulation appears to compensate for alterations in mean arterial blood pressure (157). With elevation of IOP, blood flow in the optic nerve head, retina, and choroid of cat eyes is only slightly affected before the pressure is within 25 mm Hg of the mean arterial blood pressure, and flow in the lamina cribrosa is reduced only with extreme pressure elevations, again suggesting autoregulation in the optic nerve head (158). Another study, however, suggests that the electrical function of ganglion cell axons in cat eyes depends on the perfusion pressure and not on the absolute height of the IOP (159). Real-time analysis of optic nerve head oxidative metabolism in cats indicates that the metabolic response is dependent on IOP or mean arterial pressure and that lowering the IOP can reverse metabolic dysfunction (160).
Short-term IOP elevation in monkey eyes did not alter optic nerve head blood flow until it exceeded 75 mm Hg, and long-term glaucoma in monkeys had no apparent influence on mean blood flow in the nerve head (161); others have shown that the threshold of IOP that is needed to affect blood flow is partly determined by the animal’s systemic blood pressure (162). A study of oxygen tension in the monkey optic nerve head suggested that autoregulation compensates for changes in perfusion pressure (163), and a noninvasive phosphorescence imaging technique in cats revealed well-maintained oxygen tension in the optic nerve head and retina despite increasing IOP, until blood flow to the eye was stopped (164).
Blood-flow measurements in the optic nerve head of human eyes, using laser Doppler, demonstrate autoregulatory compensation to reduced perfusion pressure secondary to elevated IOP (165). In glaucomatous eyes, however, Doppler studies show reduced flow velocity in the nerve head (166–169). Blood flow of the optic nerve head lamina, rim area, and retrobulbar flow is decreased with increasing glaucomatous damage (170,171). Eyes with glaucoma also appear to have more diurnal fluctuation of optic nerve blood flow (172).
A technique of continuously monitoring disc brightness during and after an abrupt artificial elevation of IOP also showed that the extent to which a glaucomatous eye can adjust to the pressure changes is significantly reduced from that of nonglaucomatous eyes (173). Diminished autoregulatory response to postural changes in the retinal vasculature of patients with glaucoma is also seen (174). Age may influence the vascular responses to IOP. One study showed that major retinal vessels at the disc border increased in caliber in response to IOP reduction in patients with COAG who were 55 years or younger, but not after that age (175). In children, intracranial pressure also affects optic nerve blood flow (176). It may be that ischemia of the optic nerve head in glaucoma involves faulty autoregulation, which may worsen with age and is also affected by systemic blood pressure and intracranial blood pressure (158,177,178). Molecules such as endothelin and nitric oxide are being investigated for their possible role in the normal and altered autoregulatory responses (179,180).
Fluorescein Angiography
Normal Fluorescein Pattern
The normal fluorescein pattern of the optic nerve head is usually described as having three phases (14):
In the first phase, an initial filling, or preretinal arterial, phase is thought to represent filling of the prelaminar and lamina cribrosa regions by the posterior ciliary arteries. Fluorescein in the retrobulbar vessels may also contribute to this phase (181).
The peak fluorescence, or retinal arteriovenous phase, is primarily due to filling of the dense capillary plexus on the nerve head surface from retinal arterioles. With increasing age, there is a decrease in the filling time of both the retinal and choroidal circulations (182).
A late phase consists of 10 to 15 minutes of delayed staining of the nerve head, probably because of fluorescein in the connective tissue of the lamina cribrosa. Tracer studies in monkeys suggest that the leakage may come from the adjacent choroid (183).
Effect of Artificially Elevated IOP
The effect of artificially elevated IOP on the fluorescein angiographic pattern has provided an understanding of the relative vulnerability of ocular vessels to elevated pressure in the normal and glaucomatous eyes. There is a general delay in the entire ocular circulation in response to an elevation of the IOP. The prelaminar portion of the nerve head appears to be the most vulnerable portion of the ocular vascular system to elevated pressure in monkeys (14,184).
Studies regarding the vulnerability of the peripapillary choroid to IOP elevation have provided conflicting results. Fluorescein angiography of monkey eyes has suggested a marked susceptibility of this vascular system to elevated pressure (14,184), and fluorescein studies of human eyes with glaucoma have shown similar delays in peripapillary choroidal filling (184–188). The delay appears to be sensitive to elevated IOP (185). It has been suggested that this vascular disturbance of the peripapillary choroid contributes to glaucomatous optic atrophy (187). However, fluorescein angiographic studies of normal human eyes have shown similar delayed or irregular choroidal filling at normal pressures (189,190), and the peripapillary choroidal capillaries of normal human eyes were relatively resistant to artificial pressure elevations (191). Furthermore, a fluorescein study of patients with low-tension glaucoma or COAG provided no evidence that hypoperfusion of the peripapillary choroid contributed to optic nerve hypoperfusion (192).
A selective nonfilling of the retinal radial peripapillary capillaries during India ink perfusion has been demonstrated in cats (193). As previously discussed, however, histopathologic observations differ regarding alterations of this vascular system in glaucomatous eyes (122,123). Most studies of monkey and normal human eyes have shown the choroidal circulation in general to be more vulnerable than that of the retina to elevated IOP (14,184,187,194), although one study found the two systems to fill at the same level of increased pressure (195). Regional differences in circulation of the optic nerve head, retina, and peripapillary choroid have been reported (196).
Studies of Glaucomatous Eyes
Fluorescein angiographic studies of glaucomatous and nonglaucomatous eyes have revealed two types of filling defects of the optic nerve head: (a) persisting hypoperfusion and (b) transient hypoperfusion (192,197).
Persisting hypoperfusion, or absolute filling defects, is more common in eyes with glaucoma, especially low-tension glaucoma, and are said to correlate with visual field loss (192,197,198). The characteristics of a filling defect include decreased blood flow, a smaller vascular bed, narrower vessels, and increased permeability of the vessels (199). The filling defect may be either focal or diffuse. The former is thought to reflect susceptible vasculature with or without elevated IOP, and is the typical defect in low-tension glaucoma (192). Focal defects occur primarily in the inferior and superior poles of the optic nerve head (197,198,200). In glaucomatous eyes, they are most often seen in the wall of the cup, whereas in nonglaucomatous eyes they occur more commonly in the floor of the cup (201). The diffuse defect is thought to represent prolonged pressure elevation (192).
The nature of the defect in COAG is thought to be specific, and fluorescein angiography of the optic nerve head may help to differentiate COAG from other conditions that have similar clinical changes in the optic disc (202). Computed image analysis has been used to objectively quantify fluorescein angiograms of the optic disc and has shown that increases in fluorescein-filling defect areas correlate with glaucomatous progression (203).
In patients with low-tension glaucoma, retinal arteriovenous passage times are prolonged in fluorescein angiography, possibly from the increased resistance in the central retinal and posterior ciliary arteries. Arteriovenous passage correlated with the size of the optic nerve head, visual field indices, and contrast sensitivity (204).
Axoplasmic Flow
Physiology of Axoplasmic Flow
Axoplasmic flow, or axonal transport, refers to the movement of material (axoplasm) along the axon of a nerve (the dendrite may also have transport) in a predictable, energy-dependent manner. This movement has been characterized as having fast and slow components, although numerous intermediate rates may also exist (205). The fast phase moves approximately 410 mm/day in various species and may supply material to synaptic vesicles, the axolemma, and agranular endoplasmic reticulum of the axon; the slow phase moves at 1 to 3 mm/day and is believed to subserve growth and maintenance of axons (205). The flow of axoplasm may be orthograde (from retina to lateral geniculate body) or retrograde (lateral geniculate body to retina) (206).
Experimental Models of Axoplasmic Flow
Animal models (usually in monkeys) have been developed for studying axoplasmic flow by injecting radioactive amino acids, such as tritiated leucine, into the vitreous. In other animal models, the results may have less generalizability to human glaucoma because of species differences of the lamina cribrosa region; some animals do not have a lamina. The amino acid is incorporated into the protein synthesis of RGCs and then moves down the ganglion cell axon into the optic nerve, allowing histologic study of the orthograde movement of radioactively labeled protein (207). In addition, retrograde flow can be studied by observing the accumulation of certain unlabeled neuronal components, such as mitochondria by electron microscopy (208), or by injecting tracer elements, such as horseradish peroxidase into the lateral geniculate body and studying its movement toward the retina (209). These models can be used to study factors that cause abnormal blockade of axoplasmic flow, which may relate to glaucomatous optic atrophy in the human eye.
Influence of IOP on Axoplasmic Flow
Elevated IOP in monkey eyes causes obstruction of axoplasmic flow at the lamina cribrosa and the edge of the posterior scleral foramen (206,210–215). Axonal transport in monkey eyes with chronic IOP elevation is also preferentially decreased in the magnocellular layers of the dorsal lateral geniculate nucleus, to which the large RGCs project (216). The obstruction in general involves both the fast and slow phases, and the orthograde and retrograde components (206,211,213,214). In monkey eyes, the obstruction to fast axonal transport preferentially involves the superior, temporal, and inferior portions of the optic nerve head (217). The height and duration of pressure elevation influence the onset, distribution, and degree of axoplasmic obstruction in the optic nerve head (214,218,219). The mechanism by which elevated IOP leads to obstruction of axoplasmic flow is uncertain, but there are two popular theories: mechanical and vascular.
The mechanical theory suggests that physical alterations in the optic nerve head lead to misalignment of the fenestrae in the lamina cribrosa and may result in axoplasmic flow obstruction (116,130,214). In support of this hypothesis is the observation that elevated IOP leads to blockage of axonal transport despite an intact nerve head capillary circulation and an elevated arterial pO2 (206,220). Furthermore, obstruction of axoplasmic flow has also been reported in response to ocular hypotony (211,213,221), leading some investigators to suggest that a pressure differential across the optic nerve head, whether due to a relative increase or decrease in IOP, causes mechanical changes with compression of the axonal bundles (211,213, 221,222). In the laminar portion of pig ganglion cell axons, cytoskeletal changes are seen before disruption of axoplasmic flow; the disruption of axoplasmic flow was observed to be greater in the axons of the periphery of the optic nerve, favoring a mechanical issue as the primary pathologic process (223).
In conflict with the mechanical theory is the observation that elevated intracranial pressure in monkeys neither caused obstruction of rapid axoplasmic flow nor prevented it in response to elevated IOP, despite reduction in the pressure gradient across the lamina (224). This suggests that more than a simple mechanical or hydrostatic mechanism may be involved with obstruction of axoplasmic flow in response to elevated IOP (224). Also against the simple mechanical theory are the observations that axon damage is diffuse within bundles, rather than focal, as might be expected with a kinking effect (225), and the location of transport interruption does not correlate with the cross-sectional area of fiber bundles, the shape of the laminar pores, or the density of interbundle septa (226,227).
The vascular theory suggests that ischemia at least plays a role in the obstruction of axoplasmic flow in response to elevated IOP. Interruption of the short posterior ciliary arteries in monkeys has been reported to block both slow and fast axoplasmic flow, although it did not cause glaucomatous cupping (228–230). Central retinal artery occlusion has been associated with obstruction of rapid orthograde and retrograde axonal transport (231). Furthermore, accumulation of tracer at the lamina cribrosa was inversely proportional to the perfusion pressure in cat eyes (232), and IOP-induced blockage of axonal transport was increased in eyes with angiotensin-induced systemic hypertension (233). In monkey eyes with elevated IOP, leakage from microvasculature of the nerve head has been associated with blockade of axonal transport at the lamina cribrosa (234).
Arguing against a vascular mechanism for pressure-induced obstruction of axoplasmic flow is the observation that ligation of the right common carotid artery in monkeys, which reduced the estimated ophthalmic artery pressure by 10 to 20 mm Hg, does not significantly affect the extent to which IOP elevation interrupts axonal transport (235). When obstruction to retrograde axoplasmic flow was studied in rat eyes, a direct relationship with IOP was still found, although the influence of the blood circulation was removed and the lamina cribrosa is only a single laminar sheet (209). It may be, therefore, that factors other than, or in addition to, ischemia and kinking of axons by a multilayered lamina cribrosa are involved in the IOP-induced obstruction to axoplasmic flow.
One study has found that partial constriction of axoplasmic flow may be present at the lamina cribrosa in orthograde and retrograde directions, and that accumulations of mitochondria at that level were more common in unmyelinated axons than in adjacent, myelinated axons. The authors suggested that the constriction may be a factor in glaucoma wherein IOP is not elevated (236). Endothelin-1, which produces vasoconstriction, reduces fast axonal transport in rats (237).
The effects on axoplasmic flow in the laminar region that are seen in monkeys with experimental glaucoma are similar to those seen in one of the few species to develop spontaneous glaucoma, the American Cocker Spaniel (238).
Cerebrospinal Fluid Pressure and Glaucomatous Optic Neuropathy
Anatomically, the cerebrospinal fluid (CSF) extends anteriorly in the optic nerve sheath and the subarachnoid space to the posterior aspect of the lamina cribrosa. Although IOP has been known to play a role in glaucomatous optic neuropathy, only relatively recently has there been speculation about any effect the CSF pressure may have (239,240). Studies in dogs have shown that the biomechanical effect of altering CSF pressure on the lamina cribrosa is equal to or greater than an equivalent change in IOP (241). Studies of the optic nerve architecture in human eyes have shown that the lamina cribrosa is relatively thin and bowed posteriorly in human eyes with glaucoma (40,242) (Fig. 4.8). A recent retrospective study found that the CSF pressure in patients with COAG was significantly decreased (243). In a subsequent study, CSF pressure was also lower in patients with normal-tension glaucoma and higher in patients with ocular hypertension, compared with control participants (244). A prospective study confirmed that persons with COAG have significantly lower CSF pressures than controls do; in addition, CSF pressure was lower in patients with normal-tension glaucoma than in patients with COAG (245). In this study, IOP, CSF pressure, and blood pressure were positively correlated, suggesting a dynamic interplay among these factors. Although preliminary, these studies suggest that translaminar pressure—the difference between IOP and CSF pressure—plays an important role in the pathogenesis of glaucomatous optic neuropathy.
Figure 4.8 A: Histologic section (PAS) of the optic nerve in a nonglaucomatous eye. The lamina cribrosa is indicated. B: Histologic section of the optic nerve in a glaucomatous eye. Compared with A, the lamina cribrosa is thinner and bowed posteriorly. Note the reduction in distance between the subarachnoid space, containing cerebrospinal fluid, and the laminar tissues. (Reproduced from Jonas JB, Berenshtein E, Holbach L. Anatomic relationship between lamina cribrosa, intraocular space, and cerebrospinal fluid space. Invest Ophthalmol Vis Sci. 2003;44:5189–5195, with permission.)
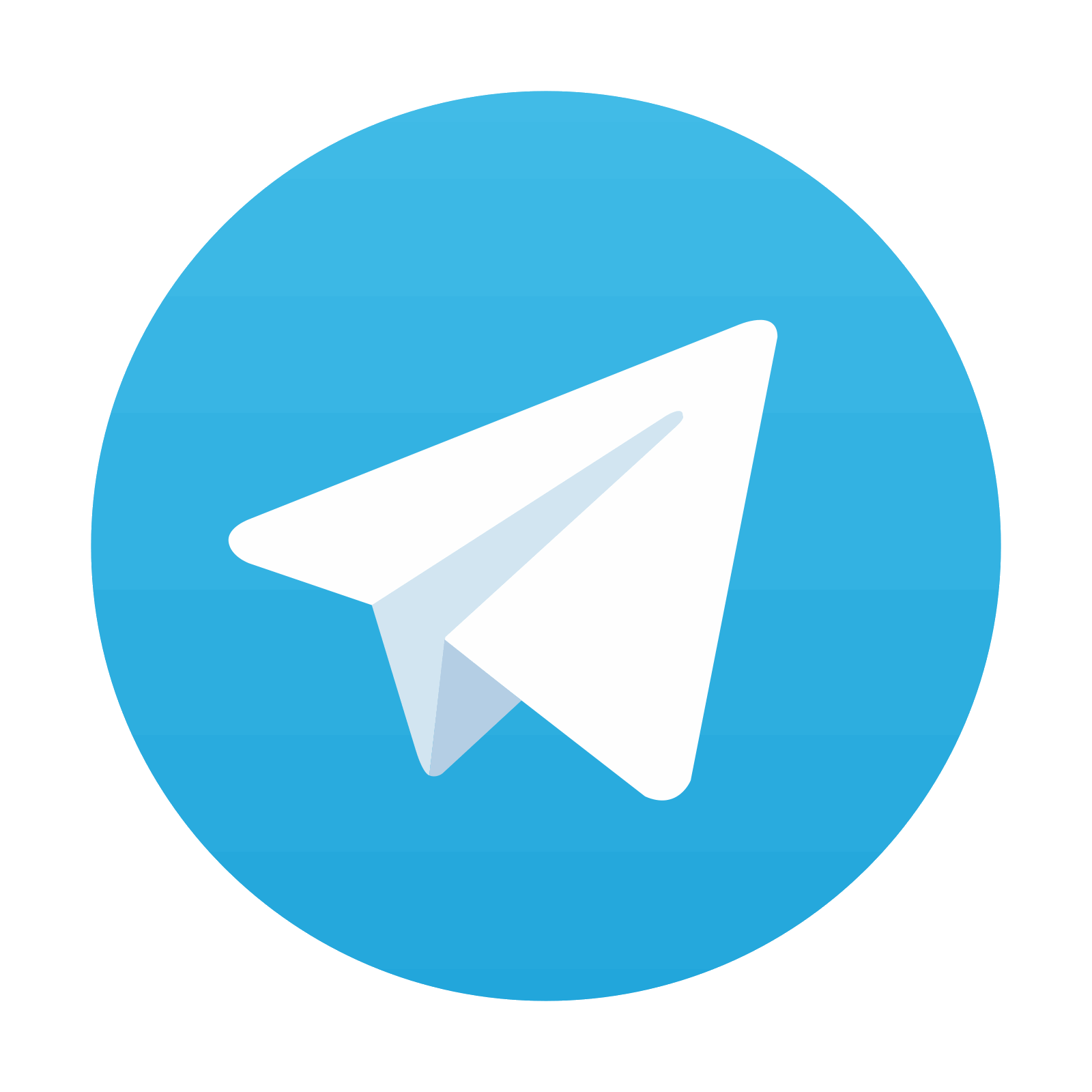
Stay updated, free articles. Join our Telegram channel
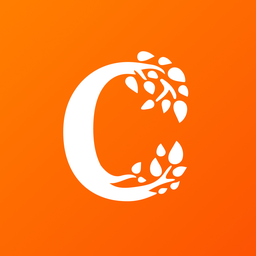
Full access? Get Clinical Tree
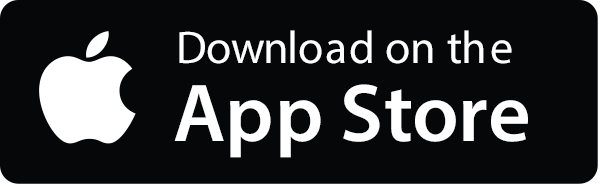
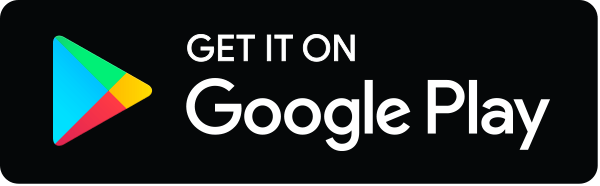