Intraocular Pressure and Tonometry
INTRAOCULAR PRESSURE
What Is Normal?
In individuals who are susceptible to glaucoma, “normal” intraocular pressure (IOP) may be defined as that pressure which does not lead to glaucomatous damage of the optic nerve head. Unfortunately, such a definition cannot be expressed in precise numerical terms because individuals show different susceptibility to optic nerve damage at given pressure levels that also depends on the underlying form of glaucoma (1,2). The best we can do is to describe the distribution of IOP in general populations to establish levels of risk for glaucoma within different pressure ranges. This chapter considers the distribution of IOP in the general population; the factors, other than glaucoma, that may influence IOP; and the clinical techniques for measuring IOP. (In Section II, the significance of various pressure levels in populations of patients with specific types of glaucoma is considered.)
Distribution in General Populations
One of the earliest studies on IOP distribution in the general population was based on Schiötz tonometry and showed an IOP distribution resembling a Gaussian curve with a skew toward the higher pressures. In 1958, Leydhecker and associates measured the IOP using Schiötz tonometry in 10,000 individuals with no known eye disease (3). The mean IOP (±standard deviation [SD]) was 15.5±2.57 mm Hg, and two SDs above the mean was 20.5 mm Hg, which the authors interpreted as the upper limit of normal because approximately 95% of the area under a Gaussian curve lies between the mean±2 SD.
Subsequent population-based and epidemiologic studies have generally agreed with the findings of Leydhecker and colleagues, and are summarized in Table 2.1. Initially these results were used to interpret two subpopulations with a larger “normal” group and a smaller group of “glaucoma” patients who had higher IOPs (Fig. 2.1). However, we now know that IOP is a causative risk factor for glaucoma on the basis of evidence from clinical trials (4–8). We also know that patients with glaucoma show different susceptibilities for disease progression at given pressure levels and based on the type of glaucoma (2). Thus, the previous simple notion that a patient’s risk for glaucoma could be determined primarily on the basis of their IOP (Fig. 2.1) is now replaced with our understanding that IOP is a quantitative trait that is influenced by many factors (9). Although it is readily measured, IOP is a complex trait determined by aqueous humor flow, uveoscleral outflow, trabecular outflow, and episcleral venous pressure (10–15) (see details in Chapter 3).
Figure 2.1 Theoretical distribution of IOPs in nonglaucoma (N) and glaucoma (G) populations, showing overlap between the two groups. Dotted lines represent uncertainty of extreme values in both populations.
Factors Affecting IOP
There have been many observations on factors that influence IOP (16–23). We should assimilate these important clinical observations from older studies with the evidence from the clinical trials, epidemiologic studies, and genetics. In addition, we should anticipate results from future studies designed to investigate the complex interactions between genetics and environment. Thus, it may be helpful to consider how these factors influence IOP on the basis of the categories of genetics, environment, and physiology.
Genetics
Early family studies provided evidence that IOP can be studied as a quantitative trait (24,25). In twin studies, IOP was observed to be more highly correlated between monozygotic than dizygotic twins (26,27). In addition, the mean IOP showed significantly higher concordance in twin-twin pairs, compared with twin-spouse pairs (27). Recently, studies have shown that heredity contributes to IOP (28–35).
Traditional genetic studies using linkage and genome-wide methods (see Chapter 8) led to the discovery of several loci, or chromosomal locations, for IOP. In the Blue Mountains Eye Study, commingling analysis of IOP supported that “a major gene” contributed to the variance of IOP (36). A family study showed significant linkage for IOP to chromosome 10q22 (37). An affected sibling pair study showed linkage to chromosomes 5q22 and 14q22 (38). In the Beaver Dam Eye Study, seven loci, on chromosomes 2, 5, 6, 7, 12, 15, and 19, were reported as being linked to IOP (39). To date, however, no “IOP genes” have been reported in these chromosomal regions. The next steps will involve validating and excluding loci, identifying genes in these loci, cross-referencing to databases, and placing these genes in context with aqueous humor dynamics. It is expected that a combination of genes will be identified as having major and minor influences on IOP variation and variation in IOP response to glaucoma medications.
Environment
Thus far, the environmental factors observed to affect IOP may be categorized into physical, smoking, drug, and dietary exposures. Exposure to cold air reduces IOP, apparently because episcleral venous pressure is decreased (40). Reduced gravity causes a sudden, marked increase in IOP, apparently because of cephalad shifts in intravascular and extravascular body fluids (41).
Tobacco smoking causes a transient rise in the IOP immediately after smoking, possibly through a mechanism of vasoconstriction and elevated episcleral venous pressure (42). However, the direct risk of tobacco on chronic open-angle glaucoma (COAG) is not evident from epidemiologic and case–control studies (43,44).
The impact of various drugs, excluding antiglaucoma drugs (discussed in Section III), are considered in the general categories of general anesthesia, illicit drugs, and systemic medications. General anesthesia is usually associated with a reduction in the IOP (45), although some agents used for sedation, such as ketamine, do not lower IOP (46). The two situations in which the physician must be particularly concerned about anesthesia-induced alterations in IOP are (a) in the evaluation of infants and children and (b) in patients who have ocular trauma with a ruptured globe.
In infants and children examined under anesthesia for suspicion of congenital glaucoma, the main concern is to avoid the artificial reduction of IOP (as discussed earlier), which could mask a pathologic pressure elevation. In one study, the mean (±SD) IOP for children measured under halothane anesthesia was 7.8±0.4 mm Hg at age 1 year, with a gradual increase of about 1 mm Hg per year of age to 11.7±0.6 mm Hg at age 5 years (47).
When operating on an open eye, such as after penetrating injury or during intraocular surgery, the primary concern is to avoid sudden elevations of IOP that might lead to extrusion of ocular contents. Depolarizing muscle relaxants, such as succinylcholine and suxamethonium, cause a transient increase in IOP, possibly because of a combination of extraocular muscle contraction and intraocular vasodilation (45). In comparing intubation methods, the laryngeal mask airway causes less of an IOP risk, compared with tracheal intubation, and has the added advantage of less postintubation coughing and other symptoms (48,49).
Among the illicit drugs, heroin and marijuana lower the IOP (the latter is discussed further in Section III), whereas LSD (lysergic acid diethylamide) elevates the IOP (50,51).
Among the many systemic medications that may potentially affect IOP, the most relevant for clinical consideration include corticosteroids, anticholinergic agents, and unusual reaction to sulfonamides. Given the use of corticosteroids systemically for immunosuppression and dramatic increased intraocular use to treat retinal diseases, the potential risk of IOP elevation and steroid-induced glaucoma should be monitored in a patient receiving such treatment (see Chapter 23).
In general, the labels on systemic anticholinergics, antihistamines, decongestants, and psychiatric medications having some anticholinergic effects state warnings such as “contraindicated in patients with glaucoma.” These warnings are meant to alert the patient and prescribing physician that use of these medications can precipitate pupillary block glaucoma or acute angle-closure glaucoma in patients with anatomically narrow angles (see Chapter 12) (52). Cases of acute angle-closure glaucoma have been reported with the use of scopolamine dermal patches for motion sickness, and the use of aerosolized atropine and ipratropium for chronic obstructive pulmonary disease (53–55). However, in patients with COAG, scopolamine was shown not to affect IOP (56). It would be expected that these other agents would not elevate IOP in patients with COAG.
The potential effects of dietary exposures on IOP have not been studied extensively (57). Acute doses of alcohol lower IOP, but the mechanism is not associated with a change in facility of aqueous outflow (58). The mechanism may be a combination of suppressed circulating antidiuretic hormone, leading to a reduction of net water movement into the eye, and direct inhibition of aqueous secretion (59). However, the clinical relevance of this acute effect is unknown since recent epidemiologic studies have not shown that alcohol consumption affects IOP or the risk for glaucoma (60–62).
Caffeine consumption may cause a slight, transient rise in IOP, although the levels associated with customary coffee drinking do not appear to cause a significant, sustained pressure elevation (63). There does not appear to be an overall population-based associated risk for glaucoma with caffeine consumption (64).
Recent epidemiologic studies have used validated nutritional surveys to analyze the association between certain dietary exposures and risk of COAG. In the Nurses’ Health Study (with 76,200 respondents) and the Health Professionals Follow-up Study (40,284 participants), no strong association was found between antioxidant consumption and the risk of COAG (65). According to a women’s health study of 1155 participants, a higher intake of certain fruits and vegetables may be associated with as much as a 69%-decreased risk of glaucoma (66). In a study comparing diets with sufficient and deficient intakes of omega-3 fatty acids since conception, those rats fed a sufficient omega-3 diet had decreased IOP with increasing age because of increased outflow facility, likely resulting from an increase in docosanoids (67).
Physiology
Sex
Overall, sex appears to have no major effect on IOP in the 20- to 40-year age-group. In older age-groups, the apparent rise in mean IOP with increasing age is greater among women than men, and coincides with the onset of menopause, whereas the increase in the standard deviation of the IOP distribution is equal between men and women in white populations (16,22). In a population-based Japanese study, IOP did not differ between women and men (68). In the Barbados Eye Study, which had a mixed population of participants, IOP was higher among women than men (69).
Age
IOP generally increases with age. Studies indicate that children have significantly lower pressures than adults do, although tonometric measurements may be influenced by the level of cooperation of the child if he or she is awake, the type of tonometer used to measure the IOP, and the general anesthetic when the child is asleep or sedated (47,70) (discussed earlier, under “Environment”).
The reported mean (±SD) IOP, by using only topical anesthesia for the tonometry, is 11.4+2.4 mm Hg in newborns and 8.4+0.6 mm Hg in infants younger than 4 months of age (47,71). In a study of 460 children between birth and 16 years of age using a noncontact tonometer, the mean IOP increased from 9.59+2.3 mm Hg at birth to 13.73+2.05 mm Hg at 3 to 4 years, with more stable measurements obtained thereafter (72). In another study, of 405 children between birth and 12 years, using the Perkins applanation tonometer, the mean IOP was 12.02+3.74 mm Hg (73). In this pediatric cohort, IOP showed a trend of increasing IOP with age (correlation coefficient [r]=0.49) that approached adult IOP levels by 12 years of age; also observed were increased IOP with hyperopia (r = 0.69) and corneal thickness measured by pachymetry (r = 0.39). IOP was inversely proportional to axial length (r = –0.1).
Results of studies in premature infants have been conflicting, with mean IOPs of 18 mm Hg in one study and 10.13 to 10.17 mm Hg in another (74,75). The tonometer may also influence the results, with mean IOP measurements in 50 supine children younger than 5 years of 5.89 mm Hg with a handheld applanation tonometer and 14.76 mm Hg with a pneumotonometer (76). In a study of 77 children (132 eyes; mean age, 1 year, 7 months; range, 1 month to 60 months), mainly with retinopathy of prematurity (107 eyes), IOP was measured by using the Perkins, Schiötz, and Tono-Pen tonometers (70). There was no significant difference between the mean IOPs obtained with the Tono-Pen and the Perkins, but the Schiötz measurements were significantly higher than those obtained with the Perkins and Tono-Pen tonometers.
In adults, the IOP distribution is Gaussian between 20 and 40 years of age (16), but tends to increase with advancing age (22). A study of 69,643 Japanese participants suggested that study design may influence the findings, in that a cross-sectional analysis showed a significant decrease in IOP with age, whereas a longitudinal analysis showed a significant increase (77). In a Malay Singapore cohort, IOP increased with age to the sixth decade, but with further increase in age there was a decrease in IOP, resulting in an inverted-U distribution pattern (78). Regression analysis showed that age, central cornea thickness (CCT), and systolic blood pressure were significant determinants of IOP in persons aged 40 to 80 years; CCT was a more important determinant in younger persons. In the white cohort of the Beaver Dam Eye Study, a population-based study of age-related eye diseases in persons aged 43 to 86 years, significant physiologic covariates on IOP with aging included systolic and diastolic blood pressures, body mass index, hematocrit, serum glucose, glycohemoglobin, cholesterol level, pulse, nuclear sclerosis, season, and time of day of the measurement (22).
In terms of aging effects on aqueous humor dynamics, studies have shown that there is reduced facility of aqueous outflow and uveoscleral outflow, and a decrease in aqueous production (79–81). Episcleral venous pressure does not appear to change significantly with advancing age (80,82).
Ethnicity
Clinical trials and population-based studies have shown that there is an increased risk for COAG among blacks, and for angle-closure glaucoma in certain Asian populations (83–85). However, with the current understanding of IOP as a causative risk factor for glaucoma and that a thin central cornea confers an increased risk for COAG, recent studies using regression analysis of multiple covariates found that black race is not an independent risk factor, although black individuals tend to have thinner corneas, greater cup-to-disc ratios, and higher IOP, which increase their risk (86). As we learn more about the biological and genomic correlates of the clinical risk factors for glaucoma, we will understand the basis of the earlier clinical observations of ethnoracial-based risk for glaucoma.
Refractive Error
In the infant eye, elevated IOP causes axial myopia as evident by buphthalmos (discussed further in Chapter 13). In older children, a positive correlation between IOP and both axial length of the globe and increasing degrees of myopia has been reported (21,87–89). Increasing IOP was also related to myopia in a study of 321 children (mean age, 9.8 years) (90). However, more recent studies have found no correlation between higher IOP and myopia in children (91,92).
In adults, it is still not known whether myopia is a risk factor for COAG. Some epidemiologic studies show no association (93), whereas other studies report a positive association between myopia and COAG (94–96). In the studies reporting an association between myopia and COAG, it is hard to know whether the higher pressures in this group reflect early glaucoma cases or a truly higher IOP distribution throughout the myopic population.
Diurnal and Postural Variation
Like many biological parameters, the IOP is subject to cyclic fluctuations throughout the day (97–99). In a study of 1062 persons middle-aged and older, the IOP was highest during the daytime (100). A study of 690 diurnal curves found that IOP peaked in the early morning for 40% of patients, and before noon in 65% (101). In a study of persons in China with (N = 59) and without (N = 67) ocular hypertension, IOP was highest in the morning (102).
More recent studies have taken into account postural variation in IOP and showed consistent elevation of IOP at nighttime (97,103), which is physiologically relevant because sleep occurs in the supine position. Whole-body, head-down tilt leads to a further increase in IOP, which correlates with the degree of inversion, is greater in glaucomatous eyes, and appears to be related to elevated episcleral venous pressure (104–106). Thus, obtaining clinical history on type of exercise—in particular, yoga and inversion—may be relevant for the patient with glaucoma. However, it is still unknown whether IOP changes induced by position contribute to optic nerve damage.
The obvious primary clinical value of measuring diurnal IOP variation is to avoid missing a pressure elevation with single readings; however, diurnal measurement is impractical in a busy clinical practice, and the logistics of obtaining the diurnal measurements is a practical concern. In any case, many physicians use a modified diurnal curve, by measuring the IOP in the office approximately every 2 hours from early morning to late afternoon or early evening. It has been suggested that measuring IOP in supine position during office hours estimates peak nocturnal IOP better than sitting measurements do (107).
In addition to trying to detect maximum IOP data as a risk for glaucoma, detecting large IOP fluctuations is also important. In a study of 64 patients with COAG and documented IOP less than 25 mm Hg over a mean follow-up of 5 years, patients were trained to perform 5 days of home self-tonometry (described later in this chapter) (108). Although mean home IOP and baseline office IOP were similar (16.4±3.6 mm Hg and 17.6± 3.2 mm Hg, respectively), the diurnal IOP range and the IOP range over multiple days were significant risk factors for progression. The risk for visual field progression within 8 years among patients with a diurnal IOP range of 5.4 mm Hg was nearly six times as high as that among patients with IOP fluctuation of 3.1 mm Hg. Baseline office IOP had no predictive value.
The physiologic mechanisms that regulate diurnal IOP variation are complex. The IOP is regulated in part by adrenocortical steroids and catecholamines (109–111). The circadian rhythm of aqueous flow also does not appear to be influenced by plasma melatonin levels (112). In terms of circadian rhythm and the four parameters of aqueous humor dynamics, the reproducible circadian rhythm of higher aqueous humor flow in the morning compared with night does not solely explain the diurnal IOP variation (98,113,114).
Exertional Influences
Straining, as associated with the Valsalva maneuver, electroshock therapy, or playing a high-resistance musical instrument, has been reported to elevate the IOP (115–117). The mechanisms include elevated episcleral venous pressure, especially with the Valsalva maneuver; uveal engorgement; and possibly, increased orbicularis tone. Of particular clinical relevance is that overweight patients may have artificial IOP elevations when measured with Goldmann applanation tonometry, because they strain to reach the instrument; this can be overcome by measuring the pressure with the patient in a relaxed position, by using a Perkins tonometer (118).
Exercise has been shown to lower IOP in persons with and without glaucoma (119). The effect of aerobic exercise on IOP lowering is observed in patients receiving topical glaucoma medication (120). There is clinical relevance for taking an exercise history. For instance, in young patients with advanced congenital or juvenile glaucoma, exercise-induced decrease in central visual acuity and reduced foveal sensitivity on perimetry may occur transiently during exercise (121). Another example is that some patients with pigmentary dispersion syndrome or pigmentary glaucoma develop exercise-induced anterior chamber pigment dispersion with IOP elevation, which can be minimized with the use of low-dose pilocarpine before exercise (122); pilocarpine causes miosis, minimizing the contact between the midperipheral iris and zonules (see Chapter 31).
Several theories on mechanisms for exercise-induced IOP reduction have been investigated and include metabolic acidosis, and hypocapnia and blood lactate levels; exercise-induced IOP changes do not appear to be related to hydration status and other serology parameters, such as plasma osmolality (123–125). Clearly, the mechanisms involved in exercise-induced IOP reduction are complex and may differ between sedentary and conditioned patients, and between young and older patients. Regardless, future research should incorporate health behaviors that include nutritional intake, body mass index and obesity, exercise, smoking, and sleep apnea (126).
Eyelid and Eye Movement
Blinking has been shown to raise the IOP by 10 mm Hg, and hard eyelid squeezing may raise it to as high as 90 mm Hg (127). Voluntary eyelid fissure widening causes an increase in IOP of about 2 mm Hg, which may relate to an increased orbital volume from retraction of the upper eyelid into the orbit (128). Contraction of extraocular muscles also influences the IOP. There is an increase in IOP on up-gaze in healthy individuals, which is augmented by Graves infiltrative ophthalmopathy (129). During strabismus surgery, especially for eyes with thyroid ophthalmopathy, the IOP has been recorded to increase to as high as 84 mm Hg (130).
Intraocular Conditions
Some intraocular conditions may lead to a reduction in IOP. In the clinical setting of anterior uveitis without angle abnormalities, IOP may be reduced slightly. It has traditionally been thought that this is because of a decrease in aqueous humor formation (131), although anterior segment inflammation has also been shown to increase uveoscleral outflow in monkeys by reducing the density of collagen type I in the extracellular matrix of the ciliary body (132). Rhegmatogenous retinal detachment may also be associated with a reduced IOP, apparently because of reduced aqueous flow, as well as a shunting of aqueous from the posterior chamber, through the vitreous and retinal hole, into the subretinal space, and across the retinal pigment epithelium (131).
Systemic Conditions
On the basis of public health relevance, the two most common systemic diseases studied for potential contributory risk for glaucoma are hypertension and diabetes mellitus. The more recent epidemiology studies find a positive correlation between systemic hypertension and IOP in Latinos, Japanese, aging men, persons of mixed African descent, the Blue Mountains Eye Study cohort, and whites in the Beaver Dam Eye Study (88,99,133–136). In contrast, hypertension was not associated with glaucoma risk in Asian Indians (137). Retinal microvascular abnormalities seen with hypertension were not associated with risk for glaucoma among white participants in the Beaver Dam Eye Study (138). The mechanisms responsible for hypertension and risk for elevated IOP and glaucoma may involve a combination of ocular pulse pressure and ocular perfusion pressure (8,139,140).
The potential influence of diabetes on IOP and glaucoma risk is unclear on the basis of epidemiology, clinical trials, and large clinical studies. In a population-based study in 3280 Malay adults aged 40 to 80 years, diabetes and metabolic abnormalities were associated with a small increase in IOP but were not significant risk factors for glaucomatous optic neuropathy (141). In the Latino cohort of the Los Angeles Latino Eye Study, presence of type 2 diabetes and a longer duration of diabetes were independently associated with an increased risk for COAG (142). In a black cohort of African ancestry, diabetes was associated with increased IOP (134). In the Rotterdam Study and among an Asian Indian population, diabetes was not a risk factor for COAG (137,143).
An earlier study in dogs reported that retrolaminar pressure (i.e., pressure surrounding the optic nerve subarachnoid space) was lower, but dependent on cerebrospinal fluid (CSF) pressure (144). The investigators stated that the translaminar pressure gradient across the lamina cribrosa varied independently of IOP, and they hypothesized that this may be important in the pathophysiology of glaucoma. Subsequent clinical studies support this hypothesis. In a case–control study involving patients who had a lumbar puncture, the opening CSF pressure in 28 patients with COAG was 9.2±2.9 mm Hg, which was significantly lower than that of the 49 controls, in whom the pressure was 13.0±4.2 mm Hg (145). Another case–control study showed a glaucoma prevalence of 18.1% in patients with normal-pressure hydrocephalus and 5.6% in controls with hydrocephalus (146). In a prospective study, CSF pressure was lower in patients with COAG than in persons without COAG, and was lower among patients with normal-tension glaucoma than among those with high-pressure glaucoma (147).
Obesity and body mass index have also been associated with increased IOP (20,22,68,148). However, the relationship between obesity and increased body mass index and risk of glaucoma is not understood (149). In Graves disease, increased rate of ocular hypertension has been reported in several studies (150–153), and one study reported that such patients have normal corneal thickness (154). Although it is logical to hypothesize that thyroid hormone has some influence on IOP, the mechanism of this hormone on aqueous humor dynamics has not been elucidated (155,156).
Some case series and case–control studies have shown an increased rate of COAG in patients with sleep apnea (157,158). In myotonic dystrophy, the IOP is markedly low, which not only may be partially due to reduced aqueous production but also may be due to increased outflow, possibly by the uveoscleral route from atrophy of the ciliary muscles (159,160). Hyperthermia has been shown to cause an increased IOP (161). Patients with human immunodeficiency virus (HIV) have a relatively low mean IOP, which correlates with low CD4+ T-lymphocyte counts and the presence and extent of cytomegalovirus retinitis (162).
TONOMETERS AND TONOMETRY
Classification of Tonometers
All clinical tonometers measure the IOP by relating a deformation of the globe to the force responsible for the deformation (163). The two basic types of tonometers differ according to the shape of the deformation: indentation and applanation (flattening).
Indentation Tonometers
The shape of the deformation with this type of tonometer is a truncated cone (Fig. 2.2A). The precise shape, however, is variable and unpredictable. In addition, these instruments displace a relatively large intraocular volume. As a result of these characteristics, conversion tables based on empirical data from in vitro and in vivo studies must be used to estimate the IOP. The prototype of this group, the Schiötz tonometer, was introduced in 1905.
Figure 2.2 Corneal deformation created by (A) indentation tonometers (a truncated cone) and (B) applanation tonometers (simple flattening)
Applanation Tonometers
The shape of the deformation with these tonometers is a simple flattening (Fig. 2.2B), and because the shape is constant, its relationship to the IOP can, in most cases, be derived from mathematical calculations. The applanation tonometers are further differentiated on the basis of the variable that is measured.
Variable Force
This type of tonometer measures the force that is required to applanate (flatten) a standard area of the corneal surface. The prototype is the Goldmann applanation tonometer, which was introduced in 1954.
Variable Area
Other applanation tonometers measure the area of the cornea that is flattened by a known force (weight) (Table 2.2). The prototype in this group is the Maklakoff tonometer, which was introduced in 1885. The division between indentation and applanation tonometers, however, does not correlate entirely with the magnitude of intraocular volume displacement. Goldmann-type tonometers have relatively minimal displacement, whereas that with Maklakoff-type tonometers is sufficiently large to require the use of conversion tables.
Noncontact Tonometer
A third type of tonometer uses a puff of air to deform the cornea and measures the time or force of the air puff that is required to create a standard amount of corneal deformation. The prototype was introduced by Grolman in 1972.
Next, we describe these various tonometers and their techniques, and compare their relative values and limitations.
Goldmann Applanation Tonometry
Basic Concept
Goldmann based his concept of tonometry on a modification of the Maklakoff–Fick law, also referred to as the Imbert–Fick law (164). This law states that an external force (W) against a sphere equals the pressure in the sphere (Pt) multiplied by the area flattened (applanated) by the external force (A) (Fig. 2.3A):
Figure 2.3 A: The Imbert–Fick law (W=Pt×A). B: Modification of Imbert–Fick law for the cornea (W+S=Pt×A1+B).
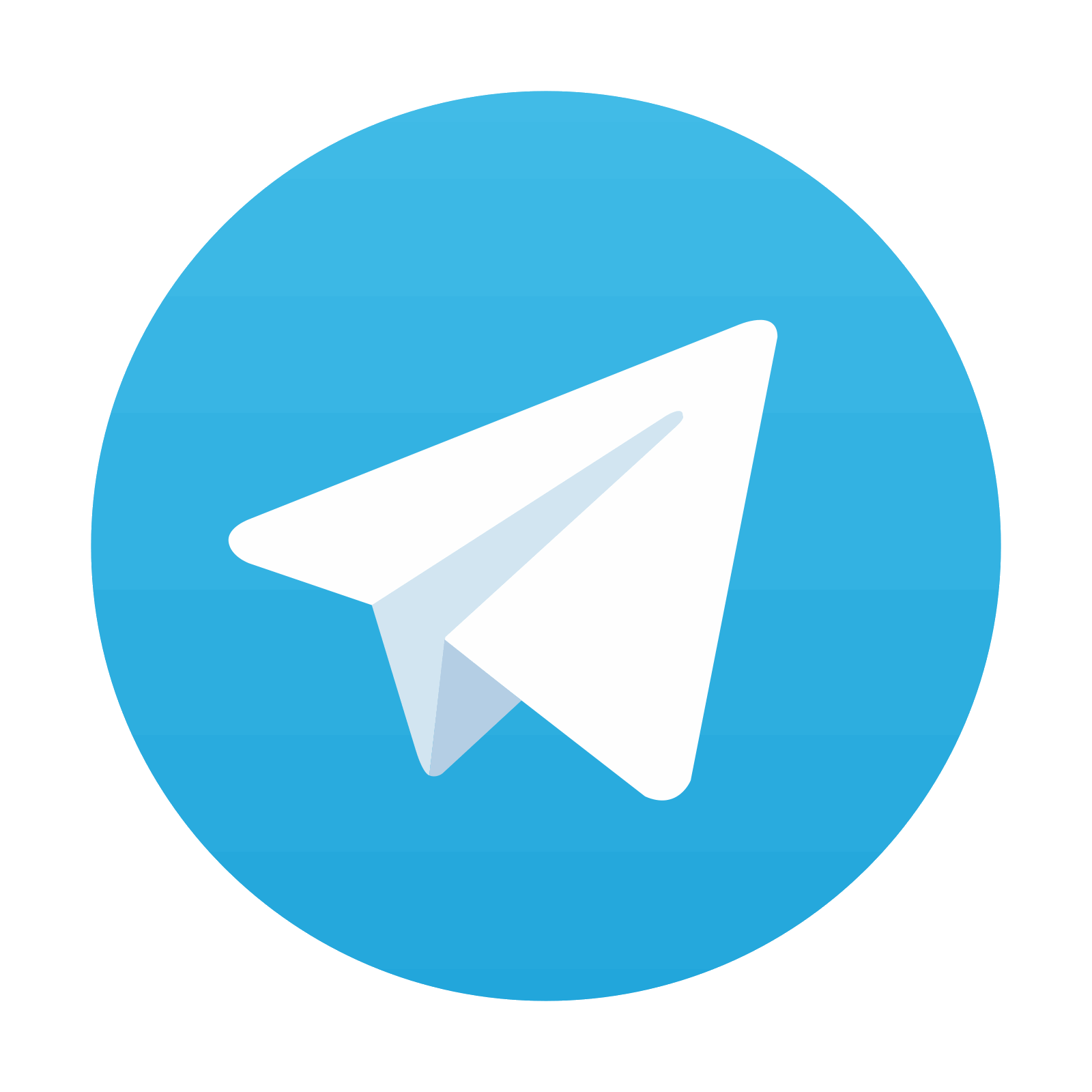
Stay updated, free articles. Join our Telegram channel
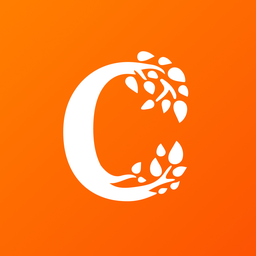
Full access? Get Clinical Tree
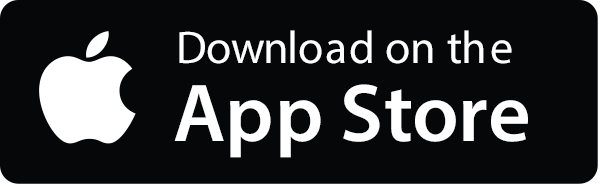
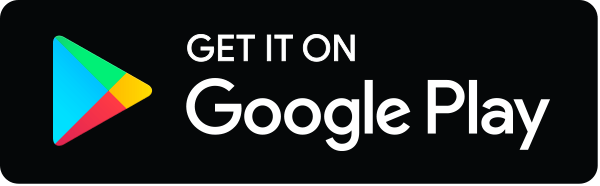