Summary
Optical coherence tomography angiography (OCTA) is an emerging technology that provides detailed images of the microvasculature of the optic nerve, peripapillary, and macular regions. OCTA of the optic nerve and peripapillary nerve fiber layer plexus shows good correlation with glaucomatous retinal nerve fiber loss and visual field defects. Both macular and peripapillary OCTA show changes consistent with changes in their corresponding structural optical coherence tomography (OCT) measures. Compared to structural OCT, macular OCTA demonstrates a deeper floor effect and thus may be useful in more severe glaucoma cases. Although this technology offers unprecedented details of the circulation, its role in the diagnosis and management of glaucoma is still unclear. Currently available commercial instruments lack the robust analytical output of its more mature structural OCT brethren, so interpreting the OCTA results in the clinical setting may be challenging. Nevertheless, OCTA can provide complementary information alongside standard structural OCT and visual field measures.
Key words
microvasculature – superficial vascular plexus – vessel density – blood flow – angiography12 Future Directions: Optical Coherence Tomography Angiography for Glaucoma
12.1 Introduction
12.1.1 The Role of Ocular Blood Flow in Glaucoma
The circulation of the optic nerve has long been thought to play an important role in the development and progression of glaucoma. However, the precise mechanism of how blood flow to the optic nerve relates to glaucomatous optic neuropathy remains poorly understood. Epidemiologic evidence indicates a strong association between lower ocular diastolic perfusion pressure, the difference between diastolic blood pressure and intraocular pressure, and prevalence of glaucoma in multiple different ethnic and geographic populations. 1 Several studies have suggested that treatment of systemic hypertension is associated with increased optic disc cupping and is a significant predictor of the development of glaucoma.
Proponents of a vascular-associated pathogenesis of glaucoma optic neuropathy propose that optic nerve head ischemia results from decreased blood perfusion, which may be due to elevated intraocular pressure and/or decreased systemic blood pressure. The increased pressure gradient between intraocular pressure and blood pressure at the optic nerve head may impede blood flow into the eye because of the increased pressure the ocular arterial system must overcome. Numerous studies have shown that ocular blood flow to the optic nerve head is decreased in eyes with glaucomatous nerve damage. 1 However, it remains unclear if the decreased blood flow is the critical factor that leads to nerve fiber loss, or if the decreased metabolic demands of the glaucomatous optic nerve results in decreased blood flow to the optic nerve head.
Prior to the development of optical coherence tomography angiography (OCTA), studies of ocular blood flow relied on technology that could only visualize or measure flow through large vessels such as the central retinal artery or the posterior ciliary arteries and their primary tributaries. 1 Because OCTA can image the microvasculature, it has become a useful tool in investigating the ocular microvasculature in glaucomatous optic neuropathy.
12.1.2 Optical Coherence Tomography Angiography Technology
Basic Technology
OCTA is an emerging technology that enables noninvasive imaging of blood vessels within the retina, choroid, and optic nerve. 2 Advancements in the acquisition speed and the depth of tissue penetration of OCT technologies have enabled the development of commercially available OCTA devices. OCTA uses the same basic technology of OCT, whereby two superimposed beams of coherent light produce an interference pattern based on the structure of the tissue being imaged. OCTA images blood vessels by detecting changes in sequential B-scan images of the same tissue. For the most part, the only difference between successive B-scans of the given tissue is the varying position of blood cells as they flow through vessels. This motion contrast is what the OCTA device interprets as blood vasculature at that particular location in the tissue (Fig. 12‑1). As with typical OCT scans, OCTA scans combine successively displaced raster B-scan data over an area of tissue to yield volumetric OCTA data (Fig. 12‑2). This enables three-dimensional representation of the microvasculature and allows segmentation of the different retinal layers and their microvasculature, as is typically visualized in standard OCT. The OCTA device then projects en face views of the microvasculature, including for several segmentation layers.


Commercial OCTA Instruments
Although all the available commercial OCTA instruments image the microvasculature using the same basic principles, they use different algorithms for signal processing and image generation. 3 Current Fourier-domain OCT systems generate signals that contain amplitude and phase information. To detect motion contrast (blood flow), algorithms are based on phase and/or amplitude information from the Fourier-transformed OCT signal, and include split-spectrum amplitude decorrelation angiography (AngioVue, Optovue, Fremont, CA), optical microangiography (AngioPlex, Carl Zeiss Meditec, Dublin, CA), and full-spectrum probabilistic approach (Spectralis OCTA, Heidelberg, Germany) (Table 12‑1).
The OCT RT XR Avanti with AngioVue detects motion contrast using split-spectrum amplitude decorrelation angiography (SSADA). The technique analyzes the amplitude of variation of the OCT signal over time for each location and calculates decorrelation. Stationary tissue will show low decorrelation (high correlation) since the signal will vary minimally over time, whereas flowing blood cells will show high decorrelation because the signal amplitude will shift widely over time as the blood cells shift in position. Blood flow through a vessel is detected if the decorrelation exceeds a threshold value.
Optical microangiography (OMAG) by AngioPlex uses both phase and amplitude information to generate motion contrast information. Phase information is extracted by measuring the phase change between adjacent B-scans, which depends on the incident angle between the direction of blood flow and the OCT beam. The Spectralis algorithm applies a probabilistic approach to detect motion contrast. The changes in OCT signal from static tissue and from flowing blood follow two distinct distributions and by analyzing multiple repeated scans (seven for the Spectralis), the algorithm determines the probability that the signal at that location corresponds to one of the two distributions.
The commercial instruments use the same optical source as their structural OCT protocols with the wavelength centered in the 840 to 880 nm range for SD-OCT or 1,050 nm for swept-source OCT.
OCTA instruments differ in the number of repeat B-scans they use to detect motion contrast, usually two or four, but seven for the Spectralis, as noted above. There is a tradeoff between acquisition speed and sensitivity when considering the optimal number of repeat B-scans. In addition, the longer acquisition times for OCTA scans make them more prone to artifacts due to bulk eye or head movement. To lessen these bulk motion signal artifacts, each manufacturer uses its own eye-tracking technology to register repeated B-scans to each other.
In addition to the modalities described above, differences among commercial OCTA devices also include time delay between repeat B-scans, algorithms to compensate for bulk motion and other artifacts, signal processing, noise reduction, and image analysis. Because of these differences, OCTA images may not be directly comparable among different instruments. 3
As with structural OCT, good signal quality is critical for proper interpretation of OCTA scan results. The commercial instruments generally use the same quality indices for OCTA scans as those used for structural OCT scans (e.g., signal strength index, signal quality).
Advantages of OCTA
Incorporated into standard OCT devices, OCTA can be performed in conjunction with standard (structural) OCT, which enables correlation between microvasculature and retinal nerve fiber layer results. Segmentation of the different retinal layers based on structural OCT enables en face visualization of different retinal capillary plexuses, including superficial or retinal nerve fiber layer plexus, deeper retinal capillary beds, and even the choriocapillaris. This is a major advantage over other vascular imaging technologies, such as fluorescein or indocyanine angiography, which presents the entire retinal vasculature en face. In addition, OCTA does not require intravenous injection of contrast dye.
Limitations of OCTA
OCTA images are prone to the same artifacts that can plague standard structural OCT images, such as decentration, segmentation error, defocus, tilt, and projection. 4 Segmentation is a particular problem because, in contrast to a structural OCT RNFL annular scan that is essentially a single B-scan cross section, the en face projection of a capillary plexus layer requires the proper segmentation across the entire scan volume of several hundred B-scans. This is particularly a problem in eyes with retinal pathology that disrupts an area of the normally organized stack of retinal layers. In addition, since OCTA requires multiple re-scanning of the same tissue plane to detect motion contrast, OCTA requires either faster imaging speeds or longer image acquisition times compared to structural OCT. Longer acquisition times leave OCTA more prone to bulk movement or blink artifacts. Overall, OCTA is more prone to artifacts than standard OCT. 4
OCTA visualizes the vascular structure, but does not measure actual blood flow, although the intensity of the vessel image may reflect the flow speed up until saturation of the image pixel. It does not detect microvasculature containing blood that is stagnant or moving too slowly to detect a change during sequential scans. As in structural OCT, OCTA scan details tend to diminish in deep structures such as the choroid and the optic nerve head deep to the lamina cribrosa.
Care should be taken when interpreting OCTA results from different instrument manufacturers. OCTA image results may not be directly comparable among different OCT devices. 3 Each OCTA manufacturer uses its own scan protocols, signal processing, data analysis, and visualization methods to render the OCTA information. The output of results also varies tremendously among the different devices. The data analysis output for OCTA is not as mature as the output for structural OCT. At the time of this writing, normative databases were not yet available for most devices and the data outputs are mostly qualitative rather than quantitative, potentially leaving the clinician at a loss for how to interpret the OCTA results or how to apply them to clinical practice.
12.2 OCTA of the Optic Nerve Head and Peripapillary Microvasculature
12.2.1 OCTA of the Optic Nerve Head Microvasculature in Normal Eyes
Since the optic nerve is the principal structure affected by glaucoma and the optic nerve head is the primary site of damage, most OCTA studies in glaucoma focus on the optic nerve and peripapillary microvasculature. The optic nerve is perfused from several arterial plexuses depending on the section of the nerve within the eye, the lamina cribrosa, and the retrobulbar region. 1 , 2 The optic nerve head prelaminar region is supplied by the choroid consisting of the scleral short posterior ciliary arteries and the recurrent choroidal arteries. These vessels enter the sclera medially and laterally and act as end arteries, which leaves the optic nerve head susceptible to ischemia. The centripetal branches from the short posterior ciliary arteries form the circle of Zinn and Haller and supply the lamina cribrosa. The retrolaminar region of the optic nerve is supplied by a peripheral centripetal system from the pial network of vessels or from an axial centrifugal supply of the central retinal artery. The primary glaucomatous changes are thought to occur in the deep optic nerve head region supplied by the posterior ciliary arteries.
Although major vessels obscure significant portions of the laminar capillaries, OCTA based on SD-OCT (840–880 nm source) can generally provide distinct images of the microvasculature within the lamina cribrosa.
The peripapillary retinal nerve fiber layer is supplied by the radial peripapillary capillary network, and this network can be well visualized by OCTA. Scan protocols of commercial OCTA instruments typically include 3 × 3 mm square en face images centered on the optic disc at three or four stacks or layers. The retinal nerve fiber layer microvasculature is usually segmented between the internal limiting membrane (ILM) and the inner plexiform layer (IPL).
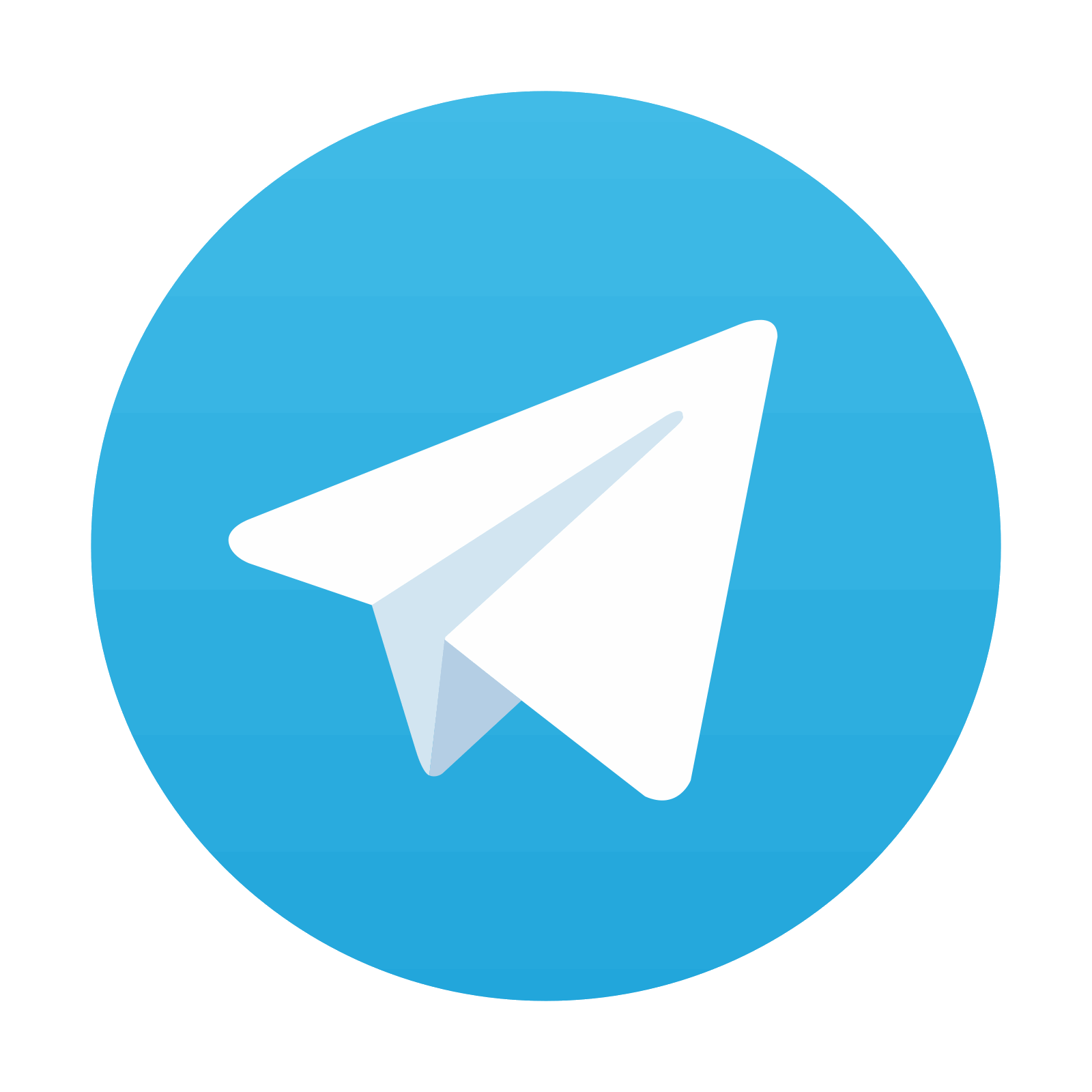
Stay updated, free articles. Join our Telegram channel
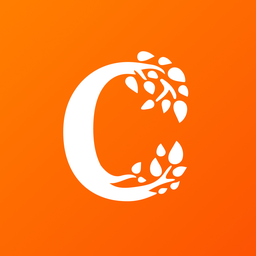
Full access? Get Clinical Tree
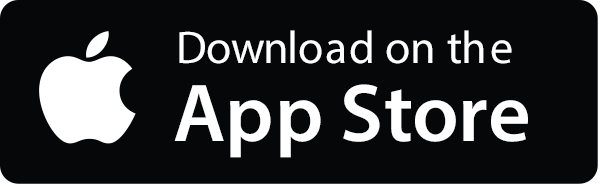
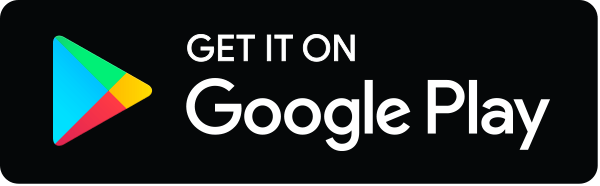
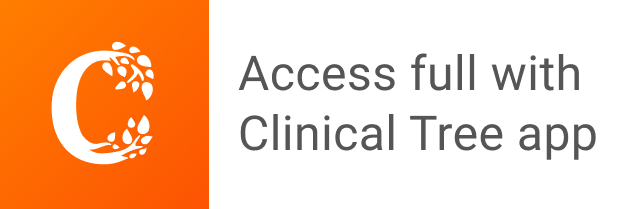