Cellular and Molecular Biology of Aqueous Humor Dynamics
The study of glaucoma deals with factors involved in the pathophysiology of progressive optic neuropathy characterized by “cupping” of the optic disc. These factors include the following disciplines: (a) clinical epidemiology, (b) clinical research and outcome studies, (c) pharmacology of glaucoma therapeutics, (d) genetics, (e) embryology and development of ocular structures, and (f) basic science investigations of the anterior and posterior segments of the ocular structures relevant to glaucoma. Because the role of lowering intraocular pressure (IOP) as a treatment of glaucoma has been substantiated by several prospective, randomized clinical trials (see Chapter 27), a logical place to begin this study is with an overview of the basic anatomy of the structural determinants responsible for aqueous humor dynamics. The basic anatomy of the optic nerve, retina, and choroid is presented in Chapter 4.
OVERVIEW OF THE ANATOMY
Aqueous humor has multiple physiologic functions throughout the various ocular structures. The two main structures related to aqueous humor dynamics are the ciliary body, the site of aqueous humor production, and the limbal region, which includes the trabecular meshwork, the principal site of aqueous humor outflow. Figure 1.1 shows the close relationship between these two structures and the surrounding anatomy.
Figure 1.1 Stepwise construction of a schematic model, depicting the relationship of structures involved in aqueous humor dynamics. A: Limbus. B: Main route of aqueous humor outflow (“conventional” or trabecular outflow). C: Ciliary body (site of aqueous humor production and other outflow route of “unconventional” or uveoscleral outflow). D: Iris and lens.
The limbus is the transition zone between the cornea and the sclera. On the inner surface of the limbus is an indentation; the scleral sulcus, which has a sharp posterior margin; the scleral spur; and a sloping anterior wall that extends to the peripheral cornea.
A sieve-like structure, the trabecular meshwork, bridges the scleral sulcus and converts it into a tube, called the Schlemm canal. Where the meshwork inserts into the peripheral cornea, a ridge is created, known as the Schwalbe line. The Schlemm canal is connected by intrascleral channels to the episcleral veins. The trabecular meshwork, Schlemm canal, and the intrascleral channels make up the main route of aqueous humor outflow.
The ciliary body attaches to the scleral spur and creates a potential space, the supraciliary space, between itself and the sclera. On cross section, the ciliary body has the shape of a right triangle, and the ciliary processes (the actual site of aqueous humor production) occupy the innermost and anterior-most portion of this structure in the region called the pars plicata (or corona ciliaris). The pars plicata region is also composed of smooth muscle, which serves the important functions of accommodation and uveoscleral outflow. The ciliary processes consist of 70 to 80 radial ridges (major ciliary processes), between which are interdigitated an equal number of smaller ridges (minor or intermediate ciliary processes) (1) (Fig. 1.2). The posterior portion of the ciliary body, called the pars plana (or orbicularis ciliaris), has a flatter inner surface and joins the choroid at the ora serrata.
Figure 1.2 Gross anatomic view of the inside view of the anterior segment showing the radial ridges of the ciliary processes at the pars plicata portion of the ciliary body.
The anterior–posterior length of the ciliary body in the adult eye ranges from 4.6 to 5.2 mm nasally to 5.6 to 6.3 mm temporally, according to various reports, with the pars plana accounting for approximately 75% of the total length. The most rapid phase of growth of the proportions of the pars plana occurs between 26 and 35 weeks’ gestation (2). At birth, these measurements are 2.6 to 3.5 mm nasally and 2.8 to 4.3 mm temporally, and they reach three fourths of the adult dimensions by 24 months, with a constant ratio between pars plicata and pars plana (3).
The iris inserts into the anterior side of the ciliary body, leaving a variable width of the latter structure visible between the root of the iris and the scleral spur, referred to as the ciliary body band. The lens is suspended from the ciliary body by zonules and separates the vitreous posteriorly from the aqueous humor anteriorly. The iris separates the aqueous humor compartment into a posterior and an anterior chamber, and the angle formed by the iris and the cornea is called the anterior chamber angle. Further details regarding the gonioscopic appearance of the anterior chamber angle are considered in Chapter 3.
With this basic outline of the anatomic structures that regulate aqueous humor dynamics, it is important to review the development of these structures and other structures of the eye. Current clinical training teaches clinicians to subclassify various ocular disease phenotypes among patients who have “outside” ocular abnormalities (or ocular phenotypes) that often have a strong genetic component, which is discussed in Chapter 8. (Another useful resource for information on human diseases with a genetic component is “Online Mendelian Inheritance in Man,” or OMIM. It can be accessed at www.ncbi.nlm.nih.gov.) As more disease genes are identified, the clinical phenotypic presentations, which are an “outside in” approach to understand disease, will merge with an “inside out” approach, whereby identified gene mutations and risk alleles are related to the ocular and systemic phenotypes. Our knowledge of the human genome, which has approximately 30,000 genes (4), and proteinomics (5), which is the study of proteins, will provide a blueprint for understanding individual variations in eye anatomy and ocular disease presentations (6).
EMBRYOLOGY OF THE EYE
The eye shows incredible diversity among the various phyla from simple eye spots, through compound eyes, to complex structures with a single lens and photoreceptor arrays (7). The developmental biology of the vertebrate eye from surface ectoderm, neural crest, and mesodermal mesenchyme has been extensively investigated (8). An overall schematic of eye development is summarized in Figure 1.3. The tissue origin of the various ocular structures is summarized in Table 1.1.
Figure 1.3 Schematic of early eye development from the optic vesicle stage (A), lens placode stage (B), and optic cup stage (C) During the optic cup stage (C), the neurogenesis of the retina proceeds in a highly regulated process with ganglion cells differentiating first, followed by the amacrine cells, bipolar cells, horizontal cell photoreceptors, and Müller (glial) cells (Modified from Traboulsi EI, ed. Genetic Diseases of the Eye; 1998:12, 15. By permission of Oxford University Press.)
Ocular development from these three tissue sources involves complex, specific cell growth, and differentiation processes, which are not fully understood. These complex processes involve carefully timed expression of various growth factors and their receptors, other signaling molecules and their pathways, transcription factors, and structural components (9). In general, the genes that regulate development can be categorized into different functional classes as follows: (a) structural genes, such as cytoskeletal components, which may be considered as “housekeeping” genes that carry out ubiquitous biochemical and structural functions; (b) regulatory genes, such as transcription factors (i.e., molecular switches that control mRNA production by other genes) and cell signaling molecules, which mainly determine specialized expression of genes; and (c) cell-specific genes encoding for specialized proteins of a particular cell type within an organ, such as the unique proteins expressed in the photoreceptors. Abnormalities in expression of the individual genes or interaction among multiple genes caused by gene mutations or altered expression can lead to congenital defects and human disease (Table 1.2).
The following regulatory genes have been grouped into large families of transcription factors: homeobox genes, zinc finger genes, and helix-loop-helix genes. Homeobox genes encode for a 60-amino acid DNA-binding element and specifically determine the target gene for a transcription factor. These genes are frequently involved in determining the regional identity of the embryo or individual fate and differentiation of cells (10). Examples of homeobox genes include the PAX family and POU domain family. The zinc finger family of genes is thought to be the most abundant of the transcription factors. These genes share a common motif of a zinc atom binding to a group of histidine and cysteine amino acids and holding together a small loop of amino acids. Examples of this gene family include the retinoic acid receptors (RAR) and retinoid X receptor (RAX), which direct the binding of retinoic acid. Mutations in these receptors have been associated with abnormal eye development (11). The helix-loop-helix family of genes is characterized by two helical DNA-binding domains held together by a special domain or region called as “leucine zipper” (12).
The role for these various structural, regulatory, and cell-specific genes in ocular development has been most extensively examined thus far in the retina, which is highly complex and only partially understood (12). Although not as extensively studied as retinal development, the anterior ocular segment, including the ciliary body and lens (13), also has important and complex roles in the development of the normal eye. The tissue origins of the ciliary epithelium, ciliary smooth muscle, and lens are listed in Table 1.1. The lens induces differentiation of ciliary epithelium at the edge of the optic cup (Fig. 1.3), and the iris develops later from the edge of the optic cup. The ciliary muscle and stroma differentiate after the ciliary epithelium is formed. It is not clear when during gestation the ciliary epithelium becomes active to secrete aqueous humor, but it is assumed to start very early after formation (14). As the IOP increases, the eye grows. It is also believed that the increase in IOP provides the force to generate ciliary folds in the ciliary body and to change the shape of the cornea (15).
Abnormalities in the development of the anterior chamber angle, or anterior segment dysgenesis, are exemplified in Axenfeld–Rieger syndrome (see Chapter 14). Thus far, genes that have been shown most frequently to cause anterior segment dysgenesis encode transcription factors that are important in early development. These transcription factors include PITX2, PITX3, PAX6, FOXC1, FOXC2, and FOXC3 (16). In transgenic mice, the cell signaling molecule, bone morphogenetic proteins, and related signaling molecules play an important role in normal development of the anterior segment (17).
An approach to study embryology of ocular structures is using data obtained through bioinformatics—a discipline that integrates the study of genes, pathways, and function. Gene expression data, also known as transcript or mRNA expression, may be gleaned in discrete ocular tissues and at various time points in development (18). Such a “global” overview of gene expression in these discrete ocular tissues enables us to hypothesize and to design studies to answer some fundamental cell biology questions about these ocular structures. By comparing and contrasting the gene expression profiles of these discrete ocular tissues at various stages of development and the impact of environmental exposures, we will understand the function of these eye structures at the cellular and molecular level (see further discussion in Chapter 8).
BIOLOGY OF AQUEOUS HUMOR INFLOW
The regulation of IOP is a complex physiologic trait that depends on (a) production of aqueous humor, (b) resistance to aqueous humor outflow, and (c) episcleral venous pressure. To reduce this highly complex and only partially understood situation to its simplest form, IOP is a function of the rate at which aqueous humor enters the eye (inflow) and the rate at which it leaves the eye (outflow). When inflow equals outflow, a steady state exists, and the pressure remains constant. The remainder of this chapter deals with these inflow and outflow parameters and their complex interrelationships with the IOP.
Cellular Organization of the Ciliary Body and the Ciliary Processes
The ciliary body is one of three portions of the uveal tract, or vascular layer of the eye; the other two structures in this system are the iris and choroid. The ciliary body is composed of (a) muscle, (b) vessels, (c) epithelia lining the ciliary processes, and (d) nerve terminals from the autonomic nervous system (Fig. 1.4).
Figure 1.4 Schematic of the three major components of the ciliary body: (1) the ciliary muscle, composed of longitudinal (LCM), radial, and circular (CCM) fibers; (2) the vascular system, formed by branches of the anterior ciliary arteries (ACA) and long posterior ciliary arteries (LPCA), which form the major arterial circle (MAC); and (3) the ciliary epithelium (CE), composed of an outer pigmented and an inner nonpigmented layer.
Ciliary Body Muscle
The ciliary muscle consists of two main portions: the longitudinal and the circular fibers (Fig. 1.4). The longitudinal fibers attach the ciliary body to the limbus at the scleral spur. This portion of muscle then runs posteriorly to insert into the suprachoroidal lamina (fibers connecting choroid and sclera) as far back as the equator or beyond. The circular fibers occupy the anterior and inner portions of the ciliary body and run parallel to the limbus. One-third portion of the ciliary muscle has been described as radial fibers, which connect the longitudinal and circular fibers. The physiologic function and pharmacologic action of parasympathomimetic agents as they relate to the ciliary muscle are discussed in Chapter 32.
Ciliary Body Vessels
On the basis of studies in primate and human eyes, the vessels of the ciliary body appear to have a complex arrangement with collateral circulation on at least three levels (19,20): (a) The anterior ciliary arteries on the surface of the sclera send out lateral branches that supply the episcleral plexus and anastomose with branches from adjacent anterior ciliary arteries to form an episcleral circle. (b) The anterior ciliary arteries then perforate the limbal sclera. In the ciliary muscle, branches of these arteries anastomose with each other as well as with branches from the long posterior ciliary arteries to form the intramuscular circle. Divisions of the anterior ciliary arteries also provide capillaries to the ciliary muscle and iris and send recurrent ciliary arteries to the anterior choriocapillaris. (c) The major arterial circle lies near the iris root anterior to the intramuscular circle and is actually the least consistent of the three collateral systems. Although the primate studies reveal a contribution from perforating anterior ciliary arteries, microvascular casting studies of human eyes, as well as several nonprimate animals, indicate that this “circle” is formed primarily, if not exclusively, by paralimbal branches of the long posterior ciliary arteries, which begin dividing in the anterior choroid. In any case, the major arterial circle is the immediate vascular supply of the iris and ciliary processes.
Each ciliary process in primates is supplied by two branches from the major arterial circle: the anterior and posterior ciliary process arterioles (20) (Fig. 1.5). Anterior ciliary process arterioles supply the anterior and marginal (innermost) aspects of the major ciliary processes. These arterioles have luminal constrictions before producing irregularly dilated capillaries within the processes, suggesting precapillary arteriolar sphincters. This may represent the anatomic site of adrenergic neural influence on aqueous humor production by regulation of blood flow through the ciliary processes. The posterior ciliary process arterioles supply the central, basal, and posterior aspects of the major ciliary processes, as well as all portions of the minor processes. These arterioles are of larger caliber than the anterior arterioles and lack the constrictions seen in the latter vessels. Both populations of arterioles have interprocess anastomoses.
Figure 1.5 Schematic of vascular interconnections of two contiguous major ciliary processes. Lateral anterior arteriolar branches join to form interprocess capillary networks (arrowhead), which provide communication between major processes. Laterally directed posterior arterioles form posterior interprocess networks through which the minor ciliary processes receive blood. In addition, both anterior and posterior interprocess networks drain directly into the choroidal veins (arrows). MAC, major arterial circle. (From Morrison JC, Van Buskirk EM. Ciliary process microvasculature of the primate eye. Am J Ophthalmol. 1984;97:372–383, with permission.)
Vascular casting studies of capillary networks in the ciliary processes of human eyes suggest three different vascular territories with discrete arterioles and venules (19). The first is located at the anterior end of the major ciliary processes and is drained posteriorly by venules without significant connections to other venules in the ciliary processes. The second is in the center of the major processes, whereas the third capillary network occupies the minor processes and posterior third of the major processes. Both of the latter territories are drained by marginal venules, which are situated at the inner edge of the major processes. It is thought that these three vascular territories may reflect a functional differentiation in the process of aqueous humor production. Venous drainage is into choroidal veins, either from the posterior aspects of the major and minor processes or by direct communication from the interprocess connections (Fig. 1.6).
Figure 1.6 Light microscopic view of ciliary processes, sectioned perpendicular to radial ridges, showing major ciliary processes and minor ciliary processes from a human eye stained with toluidine blue.
Ciliary Processes
The functional unit responsible for aqueous humor secretion is the ciliary process, which is composed of (a) capillaries, (b) stroma, and (c) epithelia (Figs. 1.4 and 1.6). The ciliary process capillaries occupy the center of each process. The thin endothelium has false “porous” areas of fused plasma membranes with absent cytoplasm, which may be the site of increased permeability. A basement membrane surrounds the endothelium, and mural cells, or pericytes, are located within the basement membrane (21).
A very thin stroma surrounds the capillary networks and separates them from the epithelial layers. The stroma is composed of ground substance, consisting of mucopolysaccharides, proteins, and plasma solutes (except those of large molecular size); very few collagen connective tissue fibrils, especially collagen type III (22); and migrating cells of connective tissue and blood origin (21). Tubular microfibrils with and without elastin have been demonstrated in bovine ciliary body, especially in the stroma of the pars plana, in relation to zonules (23).
Two layers of ciliary epithelium surround the stroma, with the apical surfaces of the two cell layers in apposition to each other (Fig. 1.7). The pigmented epithelium has numerous melanin granules in the cytoplasm and an atypical basement membrane on the stromal side.
Figure 1.7 Schematic of the ciliary epithelium summarizing the histology and junctional complexes (A), physiology of ionic transport mechanisms (B), transmembrane signaling and enzymatic pathways and other paracrine functions (C).A: The ciliary epithelium is composed of two layers containing nuclei (N) with an outer pigmented layer (facing the stroma of the ciliary process) and inner nonpigmented layer (facing and lining the posterior chamber). Apical surfaces are in apposition to each other. Basement membrane (BM) lines the bilayer and constitutes the internal limiting membrane on the inner surface. The nonpigmented epithelium is characterized by mitochondria, zonula occludens (ZO), and lateral and surface interdigitations. The pigmented epithelium contains numerous melanin granules. Additional intercellular junctions include desmosomes (D) and gap junctions (G).B: Overall, there is a net secretion (open arrows) of the cations (Na+, K+, and H+) and anions (Cl– and HCO3–), but there is also some absorption (solid arrows) of these ions. The net effect is a negative charge (⊖) toward the posterior chamber relative to the ciliary body stroma (⊕). The transfer of these ions proceeds primarily through a transcellular route, or transport across the bilayer through some ion channels and transporters (black rectangles) Transfer also occurs to a lesser extent through the paracellular route, or between the cells.C: Aqueous humor secretion is highly regulated by multiple transmembrane receptor-mediated pathways (GPCR, G-protein coupled receptor; G, G-protein; AC, adenylate cyclase; ATP, adenosine triphosphate; cAMP, cyclic adenosine monophosphate; PLC, phospholipase C; PI, phosphatidyl inositol; DAG, diacyl glycerol; IP3, inositol trisphosphate), enzymatic-mediated pathways (CA, carbonic anhydrase type II [and possibly type IV]), and specialized transporters, such as the aquaporin type I channel (AQP1), which has restricted expression in the nonpigmented ciliary epithelium. The precise localization to pigmented versus nonpigmented and orientation on apical versus basolateral surfaces are unknown for these pathways; thus, they are represented in a bilayer couplet. Other potential paracrine functions of the ciliary epithelium include secretion of small peptides (granules).
In the nonpigmented epithelium, the basement membrane is composed of glycoproteins that are immunoreactive for laminin and collagen types I, III, and IV (24). This membrane, which faces the aqueous humor, is also called the internal limiting membrane and fuses with the zonules. The nonpigmented epithelium stains less intensely than the pigmented layer for cytokeratin 18 but more so for vimentin, with the predominant distribution in the crests of the pars plicata and the posterior pars plana (25). It also stains with antibodies against S-100 protein (22). Another molecule with restricted expression in the nonpigmented cells are the water channels aquaporin-1, which is also expressed in trabecular meshwork endothelium, and aquaporin-4 (26). In transgenic knockout mice, which do not express these water channels, IOP is significantly reduced compared within the wild-type mice, whose water channels are normally expressed. The mechanism of IOP lowering is through reduction in decreasing aqueous humor production, but not in outflow. Although these genetically modified mice have a phenotype of lower IOP, patients with aquaporin-1 mutations have normal IOP (27).
A variety of intercellular junctions connect adjacent cells within each epithelial layer, as well as the apical surfaces of the two layers (28). Such junctions include gap junctions, which are expressed by the pigmented cells, the nonpigmented cells and the pigmented–nonpigmented cells, and tight junctions or zonula occludens, which are expressed between the nonpigmented cells. It is primarily the zonula occludens in the nonpigmented ciliary epithelium that creates an effective barrier to intermediate and high-molecular-weight substances, such as proteins.
Electrophysiologic studies of rabbit ciliary epithelium suggest that all of the cells in the epithelium function as a syncytium (29). Tight junctions create a permeability barrier between the nonpigmented epithelial cells, which forms part of the blood–aqueous barrier. These tight junctions are said to be the “leaky” type, in contrast to the “nonleaky” type in the blood–retinal barrier, and may be the main diffusional pathways for water and ion flow. Microvilli separate the two layers of epithelial cells. In addition, “ciliary channels” have been described as spaces between the two epithelial layers. These channels may be related to the formation of aqueous humor in that they develop between the fourth and sixth months of gestation, corresponding to the start of aqueous humor production.
The Autonomic Innervation of the Ciliary Body
Both sympathetic and parasympathetic nerve endings innervate the ciliary body (30). The sympathetic fibers synapse in the superior cervical ganglion, and the postsynaptic fibers are distributed to the ciliary body vessels. Because the ciliary epithelium is not innervated, it is thought that the catecholamine neurotransmitters released from the sympathetic nerve endings “diffuse” to the adrenergic receptors on the ciliary epithelium. Stimulation of these receptors increases aqueous humor secretion by the ciliary epithelium (discussed further in the section on Molecular Mechanisms and Regulation of Aqueous Humor Production).
The parasympathetic fibers originate from the Edinger-Westphal nucleus to innervate the ciliary muscles. Stimulation of these nerve fibers releases acetylcholine, which then stimulates the cholinergic receptors on the ciliary muscle. These activated receptors cause the ciliary muscle to contract, causing accommodation by changing the shape of the crystalline lens. In addition, ciliary muscle contraction reduces resistance to conventional aqueous humor outflow, or trabecular outflow, and may also affect unconventional aqueous humor outflow, or uveoscleral outflow. The effect of the cholinergic pathway on the trabecular outflow pathway is used pharmacologically in the treatment of glaucoma and is discussed in Chapter 32.
Molecular Mechanisms and Regulation of Aqueous Humor Production
Aqueous humor is a dynamic intraocular fluid that is vital to the health of the eye. The precise localization of aqueous humor production appears to be in the anterior portion of the pars plicata along the tips or crests of the ciliary processes (Fig. 1.2). This region has increased basal and lateral interdigitations, mitochondria, and rough endoplasmic reticulum in the nonpigmented ciliary epithelium; more numerous fenestrations in the capillary endothelium; a thinner layer of ciliary stroma; and an increase in cell organelles and gap junctions between pigmented and nonpigmented epithelia (30).
Aqueous humor is derived from plasma within the capillary network of the ciliary processes. The circulating aqueous humor enters the posterior chamber and flows around the lens and through the pupil into the anterior chamber. Within the anterior chamber, a temperature gradient (cooler toward the cornea) creates a convection flow pattern, which may occasionally be visualized clinically when a patient has inflammation with circulating inflammatory cells. Initially, to reach the posterior chamber, the various constituents of aqueous humor must traverse the three tissue components of the ciliary processes, that is, the capillary wall, stroma, and epithelial bilayer. The principal barrier to transport across these tissues is the cell membrane and related junctional complexes of the nonpigmented epithelial layer, and substances appear to pass through this structure by the following processes: (a) diffusion (lipid-soluble substances are transported through the lipid portions of the membrane proportional to a concentration gradient across the membrane), (b) ultrafiltration (water and water-soluble substances, limited by size and charge, flow through theoretical “micropores” in the protein of the cell membrane in response to an osmotic gradient or hydrostatic pressure), or (c) secretion (substances of larger size or greater charge are actively transported across the cell membrane). The latter process is mediated by transporters, which are proteins in the membrane, and requires the expenditure of energy generated by adenosine triphosphate (ATP) hydrolysis (29).
Basic Physiologic Processes
The following simplified three-part scheme describes the basic physiologic processes involved in aqueous humor production.
Accumulation of Plasma Reservoir
First, tracer studies suggest that most plasma substances pass easily from the capillaries of the ciliary processes, across the stroma, and between the pigmented epithelial cells before accumulating behind the tight junctions of the nonpigmented epithelium (30). This movement takes place primarily by diffusion and ultrafiltration. Drugs that alter perfusion of the ciliary blood vessels may exert their influence on IOP at this level (20).
Transport across Blood–Aqueous Barrier
Second, as mentioned previously, active secretion is a major contributor to aqueous humor formation (29). This active transport takes place through selective transcellular movement of certain cations, anions, and other substances across the blood–aqueous barrier formed by the tight junctions between the nonpigmented epithelium (Fig. 1.7). The process of aqueous humor secretion is mediated by transferring NaCl from the ciliary body stroma to the posterior chamber with water passively following. This secretion occurs in three steps by uptake of NaCl from stroma to pigment epithelial cells by electroneutral transporters, by passage of NaCl from pigmented to nonpigmented cells through gap junctions, and finally by release of Na+ and Cl– through Na+,K+-activated ATPase and Cl– channels, respectively.
At the first step of NaCl secretion, rabbit in vitro studies demonstrated that paired activity of Na+/H+ and Cl–/HCO–3 antiports may be the dominant mechanism in the pigmented epithelium. At the opposite nonpigmented epithelial surface, release of Na+ through Na+,K+-activated ATPase with the accompanying release of Cl– through ion channels is enhanced by agonists of A3 adenosine receptors (A3ARs). These mechanisms were confirmed in vivo in a mouse model that showed that inhibitors of Na+/H+ antiports lower IOP and that A3AR agonists and antagonists raise and lower IOP, respectively.
Carbonic anhydrase mediates the transport of bicarbonate across the ciliary epithelium through a rapid interconversion between HCO–3 and CO2 (see details in Chapter 31). Bicarbonate formation influences fluid transport through its effect on Na+, possibly by regulating the pH for optimum active transport of Na+ (31).
Other transported substances (see “Function and Composition of Aqueous Humor”) include ascorbic acid, which is secreted against a large concentration gradient by the sodium-dependent vitamin C transporter 2, or SVCT2 (32), and certain amino acids, which are secreted by at least three carriers (33).
Osmotic Flow
Third, the osmotic gradient across the ciliary epithelium, which results from the active transport of the above substances, favors the movement of other plasma constituents by ultrafiltration and diffusion. The mechanisms by which water moves from the ciliary body stroma, across the ciliary epithelium, and into the posterior chamber are complex and only partially understood. There is evidence that Na+ is the driving cationic force (29). Supporting this concept is the restricted expression of the water channels, aquaporin-1 and aquaporin-4, in the nonpigmented ciliary epithelium (26). A specific water channel antagonist has not yet been identified. The functional significance of these channels has not been extensively studied and the rare individuals with mutations of the gene encoding these water channels have a normal IOP (55).
Rate of Aqueous Humor Production
The turnover of aqueous humor within the anterior chamber is estimated to be approximately 1.0% to 1.5% of the anterior chamber volume per minute (34). The rate at which aqueous humor is formed (inflow) is measured in microliters per minute (as discussed in Chapter 2). By using the technique of scanning ocular fluorophotometry in more than 519 healthy persons, the mean (±standard deviation [SD]) rate of aqueous humor flow between 8 am and noon was 3.0 ± 0.8 µL/min (35). The normal range (i.e., 95% of the sample) was 1.5 to 4.5 µL/min and showed a Gaussian distribution of flow rates. In 490 persons, the afternoon flow rate decreased to 2.7 ± 0.6 µL/min, while the mean rate in 180 persons between midnight and 6 am was 1.3 ± 0.4 µL/min, with a range of 0.4 to 2.1 µL/min. A later study showed that individuals show concordance in aqueous humor flow, whereby those individuals who show a high aqueous flow in the morning also show a lower but relative higher flow at night (36). These changes in aqueous humor flow throughout the day reflect a biological pattern, also known as circadian rhythm, but the changes in this flow cannot account alone for the circadian patter in IOP (see modified Goldmann equation in Chapter 3) (37).
Circadian Rhythm of Aqueous Humor Flow
As noted above, there is a circadian rhythm of aqueous humor flow in humans, with rates during sleep being approximately one half of those in the morning. The mechanisms that control this biological rhythm are only partly understood and cannot be overcome entirely by light, ambulation, or activity level. The hormonal basis for the diurnal fluctuation in the rate of aqueous humor flow, or circadian rhythm, in humans is not completely understood (35). The strongest evidence suggests that physiologic changes in the level of circulating epinephrine available to the ciliary epithelia are the major driving force. Topical epinephrine has been shown to stimulate flow by 19% during the day and by 47% during the evening. Norepinephrine has also been shown to stimulate flow, but not as effectively as epinephrine. In patients who have had surgical adrenalectomy, a normal circadian rhythm of aqueous humor flow persists. In patients with Horner syndrome, where there is reduced or absent sympathetic innervation on one side, the circadian flow pattern is maintained. Systemically administered melatonin, hormones related to pregnancy, and antidiuretic hormone also do not appear to influence the normal circadian rhythm of flow. The effect of corticosteroids is more complex, in that exogenous corticosteroid appears to augment the effect of epinephrine-mediated stimulation of flow.
Other Factors Influencing Aqueous Humor Flow
Aqueous humor flow is also reduced in patients with diabetes mellitus, regardless of type (38). In myotonic dystrophy, the relative hypotony has been attributed to both reduction inflow rate and enhanced uveoscleral outflow route through the atrophic ciliary muscle (39). This causes a decrease in inflow (96), possibly related to a disruption in ciliary epithelium (97). Aqueous humor production can be reduced with inflammation (iridocyclitis) and by cyclodialysis (40).
In comparing different types of glaucoma, there are similar aqueous humor flow rates in patients with normal-tension glaucoma and healthy persons (41). Patients with ocular hypertension showed flow patterns similar to those of healthy persons during the morning hours, but the IOP and resistance to outflow values were higher in the patients with ocular hypertension (42). In patients with pigment dispersion syndrome, aqueous humor flow rate was slightly higher than in control participants because of the larger volume of the anterior chamber in the patients than in the controls (43). In patients with chronic open-angle glaucoma (COAG), aqueous humor flow during sleep was higher than in controls (44).
With aging, there is a decline in aqueous humor production—2.4% to 3.2% per decade after 10 years of age (45). There appears to be a trend of lower flow in women than in men, but this may be related to small differences in the size of the ocular structures (35). An elevation of IOP was once thought to be associated with a decline in aqueous humor production, which was referred to as “pseudofacility,” but it is now understood that aqueous humor flow is pressure insensitive (35). The osmotic stress of drinking 1000 mL of water is associated with a significant increase in aqueous humor flow after 90 minutes (46). Caffeine does not have any clinically significant effect on aqueous humor flow in the normal human eye (47).
The pharmacologic agents that reduce aqueous humor flow in the treatment of glaucoma are discussed in Section III. These agents include the β-adrenergic receptor antagonists or β-blockers (see Chapter 29), the nonspecific adrenergic and selective α2-adrenergic receptor agonists (Chapter 30), and the carbonic anhydrase inhibitors (Chapter 31).
Function and Composition of Aqueous Humor
Function
The circulating aqueous humor has at least the following functions: (a) maintaining proper IOP, which is important in early ocular development as well as in maintaining globe integrity throughout life; (b) providing substrates and removing metabolites from the cornea, lens, and trabecular meshwork; (c) delivering high concentrations of ascorbate; (d) participating in local paracrine signaling and immune responses; and (e) providing a colorless and transparent medium as a part of the eye’s optical system.
Composition
The following statements, summarized in Table 1.3, describe the general characteristics of aqueous humor, expressed relative to plasma. Aqueous humor of both the anterior and the posterior chambers is slightly hypertonic compared with plasma. It is acidic, with a pH of 7.2 in the anterior chamber (48). The two most striking characteristics of aqueous humor are (a) a marked excess of ascorbate (15 times greater than that of arterial plasma) and (b) a marked deficit of protein (0.02% in aqueous humor compared with 7% in plasma) (32,49–51).
To illustrate the constant metabolic interchanges that occur with various ocular tissues, the cornea takes glucose and oxygen from the aqueous humor and releases lactic acid and a small amount of CO2 into the aqueous humor (52). The lens takes up glucose, K+, and amino acids from the aqueous humor and generates lactate and pyruvate; however, close similarities in aqueous humor composition between the phakic and aphakic eye of the same individual suggest that lens metabolism has practically no influence on the composition of aqueous humor (53). The exchange between the vitreous and retina with aqueous humor has been shown for amino acids and glucose passing into the vitreous from the aqueous humor (33).
The relative concentrations of free amino acids in human aqueous humor vary, with ratios of aqueous humor to plasma concentrations ranging from 0.08 to 3.14, supporting the concept of active transport of amino acids (54). The concentrations of most other ions and non-electrolytes are very close to those in the plasma, and conflicting statements in the literature primarily represent differences with regard to species and measurement techniques. In general, human aqueous humor has a slight excess of chloride and a deficiency of bicarbonate and CO2 (48,55). Lactic acid is reported to be in relative excess in human aqueous humor, although this determination varies widely with the technique of measurement. Sodium in rabbits and glucose in human eyes show a relative deficiency in the aqueous humor (54).
Other molecules that have been identified in human aqueous humor may be considered potential paracrine signaling molecules (56), meaning that these molecules are circulated and distributed to local tissues. Sodium hyaluronate, a glycosaminoglycan, was reported to have a mean value of 1.14 ± 0.46 mg/g in human aqueous humor obtained before cataract extraction, with no substantial difference in patients with diabetes or glaucoma (57). Signaling molecules, such as the catecholamine, norepinephrine, and nitric oxide, have been identified in human aqueous humor (58,59). Various components of the coagulation and anticoagulation pathways may be present in human aqueous humor (60), with an overall trend toward fibrinolytic activity. Various components involved in the maintenance of extracellular matrix have been detected in aqueous humor, which may influence the trabecular meshwork activity and subsequently the IOP (61). Several growth factors, which are polypeptides involved in the homeostatic balance of cells in a tissue, have been detected in human aqueous humor, and receptors for many of these factors have been identified on appropriate target tissues, such as trabecular meshwork and cornea (56). Of interest, myocilin has been detected in normal aqueous humor, but it is absent in the aqueous humor of patients with myocilin-associated glaucoma (62).
BIOLOGY OF AQUEOUS HUMOR OUTFLOW
As noted earlier, most of the aqueous humor leaves the eye at the anterior chamber angle through the system consisting of trabecular meshwork, the Schlemm canal, intrascleral channels, and episcleral and conjunctival veins. This pathway is referred to as the conventional or trabecular outflow. In the unconventional or uveoscleral outflow, aqueous humor exits by passing through the root of the iris, between the ciliary muscle bundles, then through the suprachoroidal–scleral tissues.
The relative contribution of these outflow pathways depends on the species studied. Furthermore, there is an age-dependent change in aqueous humor outflow in both the trabecular and the uveoscleral pathways. In general, the trabecular outflow in human eyes accounts for approximately 70% to 95% of the aqueous humor egress from the eye, with the lower values corresponding to younger eyes and the higher values corresponding to older eyes (63). The other 5% to 30% of the aqueous humor leaves primarily by the uveoscleral outflow pathway, with a decline in the contribution of this pathway with age (64). Whereas both total outflow facility and trabecular outflow facility also decline with age, the relative contributions of trabecular and uveoscleral outflow show an age-related shift, with a relative increase in the contribution in the trabecular pathway. Because uveoscleral outflow is relatively independent of IOP in the physiologic range, decreased uveoscleral outflow and increased trabecular outflow resistance with age simply mean that IOP must increase sufficiently to drive a higher proportion of total flow (which remains rather constant with age) across the increased trabecular resistance.
Cellular Organization of the Trabecular Outflow Pathway
Scleral Spur
The posterior wall of the scleral sulcus is formed by a group of fibers, the scleral roll, which run parallel to the limbus and project inward to form the scleral spur (Fig. 1.1), which is composed of 75% to 80% collagen and 5% elastic tissue (65). Myofibroblast-like scleral spur cells, in close association with varicose axons characteristic of mechanoreceptor nerve endings, suggest there is a mechanism for measuring stress or strain in the scleral spur, as might occur with ciliary muscle contraction or changes in IOP (66).
Schwalbe Line
Just anterior to the apical portion of the trabecular meshwork is a smooth area, which varies in width from 50 to 150 µm and has been called zone S (67). The anterior border of this zone consists of the transition from trabecular to corneal endothelium and the thinning and termination of the Descemet membrane. The posterior border is demarcated by a discontinuous elevation, called the Schwalbe line, which appears to be formed by the oblique insertion of uveal trabeculae into limbal stroma. Clusters of secretory cells, called Schwalbe line cells, have been observed just beneath this ridge in monkey eyes and are believed to produce a phospholipid material that facilitates aqueous humor flow through the canalicular system (68).
Trabecular Meshwork
As previously discussed, the scleral sulcus is converted into a circular channel, called the Schlemm canal, by the trabecular meshwork. This tissue consists of a connective tissue core surrounded by endothelium and may be divided into three portions: (a) uveal meshwork; (b) corneoscleral meshwork; and (c) juxtacanalicular tissue, which is sometimes referred to as the cribriform layer (Fig. 1.8) (63).
Figure 1.8 Three layers of trabecular meshwork (shown in cutaway views): uveal, corneoscleral, and juxtacanalicular.
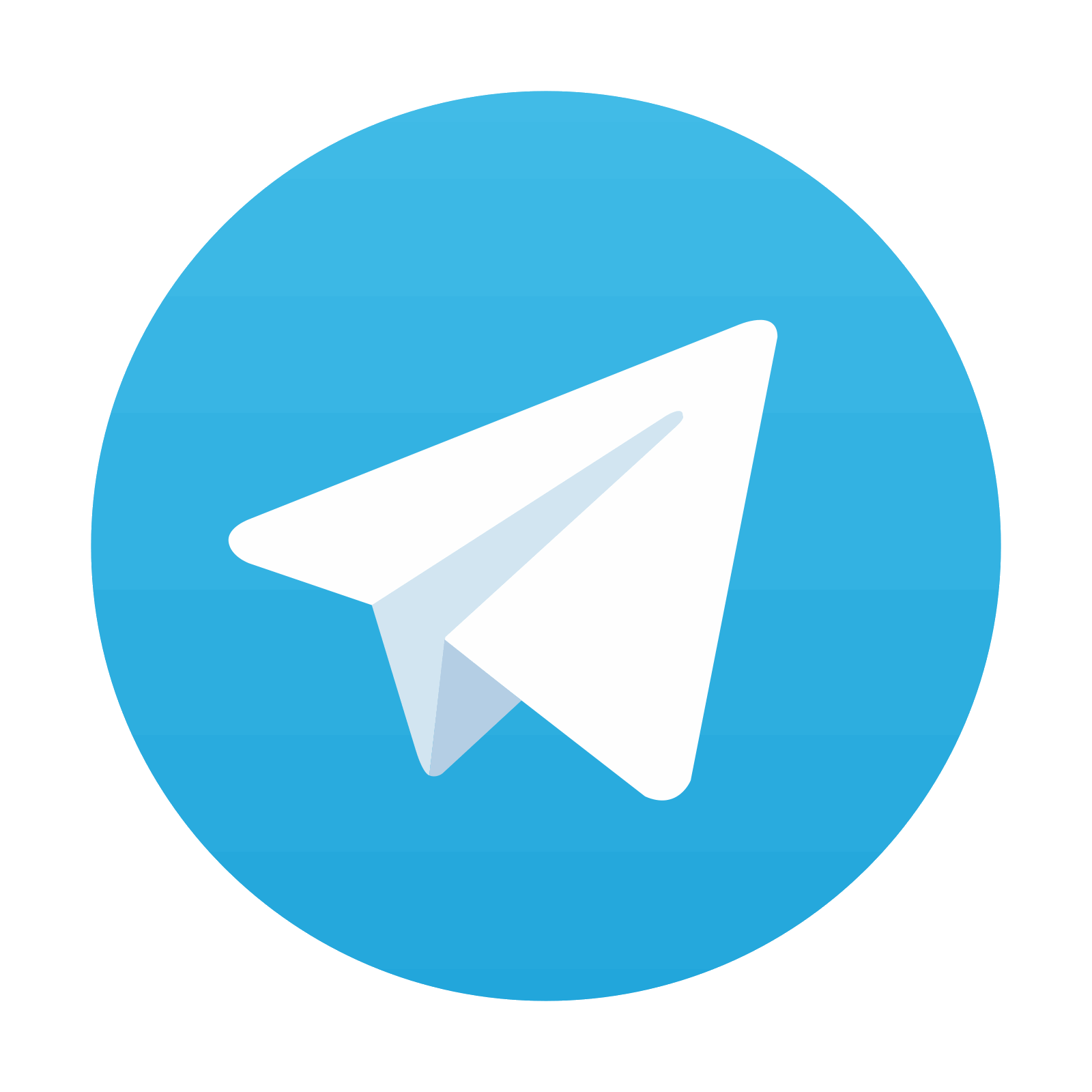
Stay updated, free articles. Join our Telegram channel
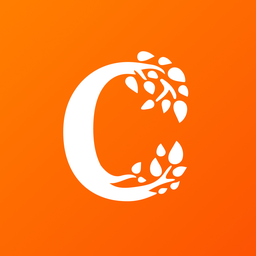
Full access? Get Clinical Tree
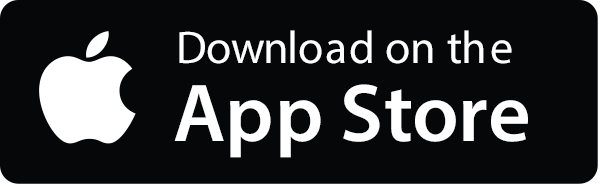
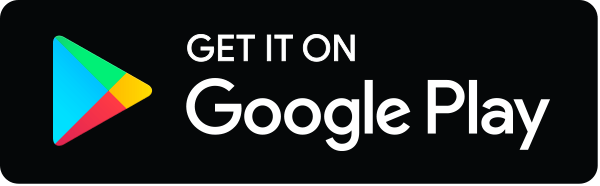