Fig. 3.1
Hand of a 22-week human fetus during fetal surgery for a life-threatening congenital cystic adenomatoid malformation associated with hydrops.
In this chapter we review basic pediatric and adult wound-healing mechanisms and then discuss those unique characteristics of scarless fetal wound healing that are known to date.
Overview of Postnatal and Adult Wound Healing
In both children and adults, wound repair proceeds in a cascade of four overlapping major stages: hemostasis, inflammation, proliferation (fibroplasia, angiogenesis, formation of granulation tissue), and scar maturation [5, 6]. In all postnatal animals (except certain amphibians), synthesis and remodeling of the new extracellular matrix in wounds lead to scarring (Table 3.1).
Table 3.1
Comparison of adult and fetal skin wound-healing characteristics
Characteristics of wound healing | Adult | Fetus |
---|---|---|
Scar and scab | Present | Absent |
Speed to closure | Slow | Fast |
Acute inflammation | Greater | Lesser |
Oxygen tension | Higher | Lower |
Sterile environment | Absent | Present |
Keratinization | Present | Immature |
Epithelialization | Slower | Faster |
Angiogenesis | Greater | Lesser |
Interleukins | Increased IL-6 and IL-8 (pro-inflammatory) | Increased IL-10 (anti-inflammatory), leads to decreased IL-6 and IL-8 |
Platelets | Higher degranulation and release of PDGF, TGF-β1, TGF-β2 | Less degranulation, aggregation, and release of PDGF, TGF-β1, TGF-β2 |
TGF-β levels | High levels of TGF-β1, TGF-β2 | Low levels of TGF-β1, TGF-β2, increased TGF-β3 |
HA/HASA | Low levels lead decreased cellular migration | Higher levels promote cellular migration and trap water |
ECM metalloproteinase | Low levels favor accumulation of collagen | Higher levels promote matrix turnover and remodeling |
Matrix deposition | Slower, disorganized | Faster, organized |
Collagen content | Predominance of type I: provides tensile strength and rigidity | Predominance of type III: promotes cellular migration and regeneration |
Fibronectin | Late deposition: disorganized tissue structure | Early deposition: organized tissue structure |
Tenascin C | Late appearance | Early appearance |
Decorin | High, binds and entraps growth factors | Low, growth factors appear transiently and are not stored |
Adhesion proteins | Low levels, leading to slow cellular migration | Rapid upregulation, leading to faster cellular migration |
Stem cells (progenitor cells) | Absent | Inherently present, capable of regenerative repair |
Integrin upregulation | Slower | Faster |
Hemostasis is the first phase of the postnatal or adult wound-healing response. Whether caused by a scalpel or burn, tissue injury leads to vascular disruption and extravasation of red blood cells, platelets, and plasma. Tissue thromboplastin and activated factor XII cause activation of the coagulation cascades and formation of a hemostatic platelet-fibrin plug (Fig. 3.2). Within the wound plug, aggregated platelets become activated after binding to fibronectin, collagen, and other wound proteins [7]. Platelets then degranulate and release proteases, vasoactive amines, and adhesive glycoproteins, such as fibrinogen and fibronectin. In addition, platelets release a cadre of biologically active growth factors, such as platelet-derived growth factor (PDGF), transforming growth factor-beta-1 (TGF-β1), and insulin-like growth factor-1 (IGF-1). Aside from limiting blood loss, the platelet-fibrin clot also forms a protective scab. Furthermore, fibrin and fibronectin within the clot form a provisional matrix that provides a scaffold for monocyte, fibroblast, and keratinocyte deposition [8].


Fig. 3.2
Early phases of wound healing : Hemostasis and inflammation. Growth factors thought to be necessary for cell movement into the wound as shown. Abbreviations: Transforming growth factor β1, −β2, and –β3 (TGF-β1, TGF-β2, TGF-β3), transforming growth factor-alpha (TGF-α), fibroblast growth factors (FGF), platelet-derived growth factor (PDGF), platelet-derived growth factor-AB (PDGF-AB), platelet-derived growth factor-BB (PDGF-BB), insulin-like growth factor-1 (IGF-1), and keratinocyte growth factor (KGF) (Figure reproduced with permission of TICEBA GmbH. Source: http://www.ticeba.com)
In the inflammatory/migratory phase of adult repair, white blood cells enter the area of injury [5, 9]. Neutrophils are recruited first via chemotactic stimulus to scavenge cellular debris, dirt, and bacteria. Neutrophils are the most predominant cell type during the first 48 h after injury in adults (Fig. 3.2). However, it has been shown that the absence of neutrophils does not prevent healing. In fact, mice studies have shown that neutrophils are not essential to the wound-healing process [10]. The expression of adhesion molecules is upregulated to facilitate passage of leukocytes from the blood stream into the wound [11]. Subsequently, cellular degradation products attract monocytes to the wound, and, along with macrophages, they synthesize a plethora of growth factors [PDGF, TGF-β, fibroblast growth factors (FGF), and transforming growth factor-alpha (TGF-α)]. These growth factors attract and activate local fibroblasts, endothelial cells, and epithelial cells to initiate their respective roles in the wound-repair process. As opposed to neutrophils, tissue macrophages are essential in wound repair. Not only do macrophages remove debris, they also secrete collagenases and other enzymes that break down necrotic tissue [12]. Macrophages provide the signaling cascade that initiates angiogenesis and fibroblasts to regenerate the dermis. Further, macrophages can and do produce many of the matrix elements and matrix-remodeling metalloproteinases [13, 14].
In the proliferative and synthetic phases of wound healing, growth of new tissue occurs. During this phase, granulation tissue formation, angiogenesis, epithelialization, and fibroplasia restore tissue integrity and function. Within hours of skin injury, epidermal cells at the wound margin acquire a flattened phenotype and pseudopodia in preparation for wound contraction and migration across the tissue defect [15]. In addition, migrating keratinocytes upregulate expression of specific cell surface receptors that bind to extracellular molecules within the wound matrix, such as hyaluronic acid, fibronectin, fibrin, and collagens [16–18]. Growth factors regulate reepithelialization [5, 15, 19]. TGF-β and epidermal growth factor (EGF) stimulate keratinocyte migration and synthesis of fibronectin. EGF and TGF-α appear to mediate epidermal cell proliferation at the wound margin [20]. Once epithelial integrity is restored, basement membrane proteins (laminin, collagen) reappear, and keratinocytes reform hemidesmosomes and other cell-cell and cell-matrix attachments.
Angiogenes is is the formation of new blood vessels, or neovascularization. It occurs in concert with reepithelialization and granulation tissue formation [5, 9, 21]. Endothelial cells at the tip of injured capillaries form new capillary buds and migrate into the wound in response to several factors (fibronectin, heparin, PDGF, basic FGF, and vascular endothelial growth factor (VEGF)). Low oxygen tension, lactic acid, and biogenic amines further potentiate angiogenesis. Basic FGF and heparin act in synergy to enhance new vessel growth.
Within 48–72 h after injury, granulation tissue begins to form during the proliferative phase of wound healing. Granulation tissue consists of a tissue matrix hosting a variety of cell types. Fibroblasts from the surrounding tissue migrate into the wound, proliferate, and begin to synthesize extracellular matrix molecules by excreting collagen and fibronectin [5, 21]. Macrophage-derived growth factors , such as TGF-β, stimulate fibroblast proliferation and increased synthesis of collagen, elastin, and proteoglycans. During wound remodeling, fibroblasts secrete hyaluronidase to break down and replace the provisional hyaluronate-rich matrix with larger sulfated glycosaminoglycans. With further wound remodeling, type I collagen gradually replaces type III collagen as the primary collagen in scarring.
Subpopulations of fibroblasts may serve different functions in the wound-repair process. Myofibroblasts are specialized mesenchymal cells that share functional and structural characteristics of both fibroblasts and smooth muscle cells. They express a type of actin called α-smooth muscle actin , a cytoskeletal microfilament that functions in smooth muscle type contraction using the myosin-actin complex [22–24]. Myofibroblasts are naturally found in some subepithelial mucosal surfaces such as the gastrointestinal or genitourinary tract. They provide support and have paracrine function. They were first described in granulation tissue and appear transiently to mediate wound contraction, collagen synthesis, and scar formation. They help speed up wound repair by contracting the edges of the wound. After wound healing is complete, and during the maturation phase, collagen is realigned along tension lines, and myofibroblasts undergo apoptosis; however they may occasionally persist and contribute to the formation of keloids or hypertrophic scars. Myofibroblasts additionally persist in many fibrotic diseases, such as hepatic cirrhosis, renal and pulmonary fibrosis, Dupuytren contracture, and neoplasia-induced desmoplastic reactions. Understanding the signals that regulate myofibroblast apoptosis may contribute to our understanding of hypertrophic scars and how to prevent their formation.
In newborn and adult animals alike, matrix synthesis and remodeling invariably lead to scar formation [5, 9, 21]. Although scar remodeling occurs for months to years after the initial injury, complete restoration of the normal extracellular matrix architecture is never achieved [6]. Mature scars restore only 70% of the tensile strength of normal skin, and normal function is never completely recovered [25]. In general, scarring refers to alteration in the normal composition and organization of a tissue. Scarring is perhaps best characterized in skin, where it refers to a disruption in the pattern of collagen and other extracellular proteins of the dermis. A mature scar is a relatively acellular, avascular area with abundant parallel bundles of collagen, a lack of elastin, and an absence of dermal appendages such as sweat glands and hair follicles. The size of a scar depends on the extent of tissue injury, the degree of wound contamination, the inflammatory response, and the overall nutritional status of the host [9]. A clean surgical incision in which the wound edges are carefully reapproximated heals with minimal scarring. Larger, contaminated wounds result in larger scars.
In contrast, the embryo and early gestation fetus can heal skin wounds without scarring and with regeneration of dermal appendages and restoration of a normal pattern of collagen and extracellular matrix that is indistinguishable from the surrounding, unwounded skin. Although many differences between fetal and adult wound repair have been described, the key mechanisms that mediate scarless fetal repair remain unclear. In the next sections of this chapter, we review those unique characteristics of the fetus that may contribute to scarless repair as well as some new advents in the field.
Fetal Skin and the Fetal Environment
Several fundamental differences exist between fetal and adult skin that may be at the origin of the scar-free fetal tissue repair. Understanding skin embryogenesis can provide insights into the major differences between fetal and adult scarring. In the human embryo, a primitive epidermis first appears at gestational day 20. During 4–8 weeks of gestation, the human primitive epidermis develops into two layers, the periderm and the basal cell layer. Epidermal stratification begins by 9 weeks, and epidermal keratinization begins by 21 weeks. By 16 weeks, fetal skin has many of the adult skin components such as a basal cell layer, an intermediate layer, sweat glands, hair follicles, and follicular keratinization. By 24 weeks gestation, human fetal skin has all the features of mature adult skin, and at birth neonatal skin is histologically indistinguishable from adult skin [26–28].
Scarring in adult wounds occurs mainly at the dermal level. The fetal dermis similarly undergoes morphologic and biochemical changes during the healing process however without major structural alterations [26]. Early in gestation, the fetal dermis is thin and cellular with a paucity of extracellular matrix. As development proceeds, dermal collagen is deposited, and sulfated glycosaminoglycans replace hyaluronic acid and other non-sulfated glycosaminoglycans. The rapid growth rate of fetal skin and the loose, hyaluronic acid-rich composition of the extracellular matrix provide a conducive environment for scarless fetal repair. Scarless fetal wound healing was first described about three decades ago [29]. It was demonstrated by the repair model of full-thickness eyelid colobomas produced in midgestational fetal sheep (Fig. 3.3). The same occurs in humans before 24 weeks GA [2].


Fig. 3.3
Scarless repair of a fetal sheep eyelid. (a) Full-thickness left upper eyelid coloboma (one-half lid length) produced in 90-day-midgestational fetal sheep. (Observe fetal head protruding from maternal uterus.) (b) Repaired eyelid coloboma before fetal head replacement into maternal uterus. (c) Repaired eyelid coloboma at term. (d) Masson’s trichrome stain of repaired eyelid coloboma. Note normal follicles and muscle architecture without any evidence of scarring (Courtesy of Michael A. Johnson, MD, James A. Katowitz, MD, and Timothy G. Crombleholme, MD)
It has been suggested that differences between the fetal and adult wound environments may influence wound repair. Fetal wounds are bathed in warm, sterile amniotic fluid that is rich in growth factors and certain adhesion molecules, such as fibronectin and hyaluronic acid [30]. Furthermore, fetal subcutaneous tissue is relatively hypoxemic as compared to the adult counterpart. However, early evidence suggests that the fetal environment is neither sufficient nor necessary for scarless fetal repair as fetal skin outside the uterine environment also heals without scar as demonstrated by experiments on the newborn opossum [31, 32]. At birth, the opossum remains fetal-like, both physiologically and anatomically, and remains attached to the mother’s nipple for 4–5 weeks. Despite their extrauterine location, incisional wounds in early pouch young reepithelialize within 2 days, synthesize collagen within 24 h, and heal scarlessly [31]. In contrast, wounds in older pouch young heal more slowly and with scarring. Furthermore, human fetal skin transplanted subcutaneously to an immuno-incompetent mouse, such as the athymic nude mouse, retains its developmental characteristics [27] and heals scarlessly [33]. Thus, scarless repair capabilities appear to be intrinsic to the fetal tissue itself, rather than dependent on the sterile, in utero amniotic environment. On the other hand, adult skin in the fetal environment heals with scarring. When adult sheep skin is transplanted into 60-day-gestation fetal sheep (before term which is 145 days and before the fetal immune system has developed sufficiently to reject the allografts), incisional wounds in the adult grafts heal with scarring [34]. Thus, scar formation appears to be intrinsic to adult skin, and neither the amniotic fluid environment nor perfusion by fetal blood prevented scarring. Late gestation fetuses have been observed to heal with scarring despite remaining within the amniotic environment. Primate fetuses demonstrate these developmental changes in their repair response [35]. Full-thickness, upper lip wounds in fetal rhesus monkeys at 75 days GA (165 days being full term) heal scarlessly with regeneration of hair follicles and a dermal collagen pattern that is indistinguishable from unwounded tissue (Fig. 3.4). Conversely, similar wounds in fetuses at 107 days GA or older heal with scarring. Interestingly, wounds made in 85–100 day gestation fetuses heal with intermediate, transitional repair morphology. Fetal wound-healing studies in rodents [36], opossum [32], and sheep [37] demonstrate a similar developmental transition from scarless to scar-type repair. Thus, there is more to the mechanisms of scarless fetal repair than the sterile, growth factor-rich amniotic environment.


Fig. 3.4
Scarless repair of a lip wound in a fetal rhesus monkey. (a) A full-thickness upper lip wound was made in a midgestation fetal rhesus monkey. (The back and hindquarters of the fetus remain within the uterus. Babcock clamps secure the fetal membranes at the cut edge of the uterus). (b) Scarlessly healed fetal wound 14 days later. The healed wound is marked by sutures but otherwise is indistinguishable from the surrounding tissue
Not all fetal organ systems heal scarlessly. Whereas fetal skin, bone, and cartilage can regenerate, fetal nerve tissue, diaphragm, stomach, and peritoneum scar [30, 38–41]. Additionally, scarring is positively correlated with wound size, and while small fetal wounds heal scarlessly, large fetal wounds scar [42]. The unique characteristics of fetal tissue repair appear to be intrinsic to fetal skin and the cells and extracellular matrix that compose it.
Characteristics of Fetal Wound Healing
Fetal-type wound healing is most remarkable for its regenerative speed and the absence of scarring, even at the histologic level. All primary histologic structures (hair follicles and sweat glands) are restored in the process. Studies reveal that the differences between fetal and adult type of wound repair lie mainly in the inflammatory and proliferative phases [33]. Additionally, differences are noted both at the intracellular level and extracellular level/matrix (Table 3.1).
At the Cellular Level
Hemostatic Plug and Platelet Function
Differences between fetal and adult platelets may contribute to differences in the wound inflammatory response. Platelet counts, structure, alpha granules, and other organelle content are similar between fetus and adult pigs [43, 44]. However, fetal platelets show decreased aggregation to ADP and collagen compared to older fetuses or adults [43]. Additionally, serum growth factor levels in the youngest fetuses were approximately one-third to one-half those in the adult. Since nearly all serum PDGF and 90% of TGF-β is derived from platelets, a more limited inflammatory response might result from less platelet aggregation or a limited release of platelet-derived cytokines at the wound.
Inflammatory Phase and Cellular Mediators
The fetal immune system is functionally immature relative to its adult counterpart [45–49]. Fetal cells are in fact capable of mounting an inflammatory response; however this response is not elicited in the repair of early fetal wounds, and the reason behind this remains unclear. Immunoglobulin deposition is absent in lamb and mouse fetal wounds [37, 50]. Lymphocytes and polymorphonuclear leukocytes are conspicuously absent in fetal rabbit wounds [46, 51]. However, when polyvinyl alcohol (PVA) dipped, adult implanted sponges are subsequently transplanted subcutaneously into fetal rabbits, they will induce fetal neutrophil migration [48]. This suggests that adult wound chemoattractants can induce fetal leukocyte migration to the wound site. Similarly, when bacteria (live or irradiated)-rich sponges are implanted subcutaneously in fetal rabbits, or when turpentine (solvent) or carrageenan (food additive) is injected into the skin of fetal lambs, neutrophils, macrophages, and lymphocytes are recruited to the wound [52, 53].
Macrophages
In adults, macrophages synthesize matrix metalloproteinases and their inhibitors as previously described, and coordinate wound healing. The acute inflammatory infiltrate in the fetal wound consists mainly of macrophages and almost no leukocytes or lymphocytes [54], and hence the low phagocytic activity leads to less neutrophilic infiltration. Wound macrophages participate in coordination of fetal repair as they do in the adult [5, 9, 55]; however they are present in lower numbers than in the adult [56]. Fetal macrophages express a number of growth factors, just as adult macrophages do [57]. Because fetal skin repair is characterized by the restoration of a normal extracellular matrix, an essential regulatory role for fetal macrophages seems likely.
Fetal Fibroblasts
The fetal fibroblast deposits collagen and other extracellular matrix in an organized, scar-free pattern and likely plays a leading role in scarless wound repair. Therefore, it is crucial to compare the early-gestation fetal fibroblast to the late-gestation and adult fibroblast to uncover differences between fetal and adult wound-repair mechanisms. In vitro, fetal fibroblasts demonstrate several fundamental differences when compared to adult fibroblasts with regard to collagen production and the response to and production of TGF-β. Human fetal fibroblasts demonstrate greater prolyl hydroxylase activity, greater collagen synthesis [58], and higher levels of TGF-β than adult fibroblast cells in normoxic conditions [59]. However in response to hypoxia, fetal fibroblasts decrease TGF-β transcription, as opposed to adult fibroblasts. In addition, fetal fibroblasts increase synthesis of both type I and type III collagen in response to TGF-β2 as opposed to adult cells [60]. Whereas adult and neonatal fibroblast migration is stimulated by TGF-β1, human fetal fibroblast migration appears to be inhibited [61]. Studies on fetal rabbit fibroblasts suggest that TGF-β receptor density decreases with advancing gestational age [62]. In addition, fetal rabbit fibroblasts have a two- to fourfold greater number of cell surface hyaluronic acid receptors (CD44) than adult cells [63]. Thus, there appear to be fundamental differences between fetal and adult fibroblasts in the regulation and synthesis of collagen, their response to growth factors, and the expression of cell surface receptors.
At the Extracellular Level
Hyaluronic Acid
Proteoglycans are a heterogeneous group of large, polyanionic molecules that consist of a protein core covalently bound to one or many polysaccharide side chains (glycosaminoglycans). Proteoglycans likely play an important role in fetal repair. Hyaluronic acid (HA), a high molecular weight glycosaminoglycan, is present in high concentrations during embryogenesis and during periods of rapid tissue proliferation. Because of a large number of hydrophilic residues, HA gives fetal tissue a loose, porous nature. The extracellular matrix of fetal skin is rich in glycosaminoglycans, of which nearly 95% is hyaluronic acid [64]. With advancing fetal age, HA and total glycosaminoglycan content decreases, whereas the relative concentrations of sulfated glycosaminoglycans, including dermatan sulfate, heparan sulfate, and chondroitin sulfate, increase [64–66]. Along with a decrease in HA, there is a similar decrease in the water content of fetal skin, from nearly 93% in fetal lambs at 90 days gestation to 76% by term [63]. Meyer and Stern have measured total HA content in human skin from 28 weeks fetal gestation through 88 years of age [67]. Unlike previous reports, they found that the actual HA content was similar in all age groups but that HA was less bioavailable with advancing age, presumably from increased association with HA-binding proteins. Changes in glycosaminoglycan composition and the flexibility/pliability of the extracellular matrix, in association with a higher number of HA receptors on fetal fibroblasts, may account for differences in the ability of fetal cells to move efficiently through the wound environment and to effect rapid repair. Hyaluronic acid accumulates rapidly in both fetal and adult wounds or PVA sponges. In adults these levels rapidly return to baseline after 3 days, but in the fetus, elevated levels persist until 21 days after the insult [68]. Unlike adult repair, these sustained fetal HA levels are associated with elevated levels of hyaluronic acid-stimulating activity and low levels of hyaluronidase [69, 70]. A prolonged presence of HA, facilitated by fetal and amniotic hyaluronic acid-stimulating activity and low levels of hyaluronidase, provides an environment that is conducive to scarless repair.
Collagen
Collagen comprises nearly 77% of the fat-free dry weight of the skin [71]. There are differences in the content and collagen composition between fetal and adult skin and between fetal and adult wounds. In fetal skin, total collagen content increases with advancing gestational age and increased skin development. Human fetal skin collagen content more than triples between 19 and 40 weeks gestation [72]. Early-gestation fetal skin has a predominance of type III collagen rather than late-gestation fetal or adult skin. In humans the ratio of type I to type III collagen is nearly 1 at midgestation and 2.7 at term [73]. This preponderance of type I collagen persists throughout postnatal life [73]. Scar tissue is morphologically represented by disruption of the normal dermal spatial composition of collagen. Since type III collagen has a smaller, reticular structure and has a greater propensity to cross-link than type I collagen, it may contribute to the regeneration of the fine collagen network after wounding. Type I collagen in postnatal wounds provides more strength and rigidity to the skin; however this may impede the regenerative process by haltering cellular migration. Similar to unwounded skin, scar tissue shows a difference in its collagen composition, whereby collagen type I is predominant in adult wounds and type III is predominant in fetal wounds [74]. Rapid fetal repair and collagen deposition have been demonstrated in multiple animal models [2, 37, 50, 56]. Nath and colleagues compared the levels of type I collagen expression between fetal and adult rabbits [75]. Whereas fetal wounds showed increased collagen expression by 3 days after wounding and only a modest increase in wound cellularity (1.5-fold), adult wounds did not show increased collagen expression until 7 days after wounding, and there was a much greater early increase in wound cellularity (6.3-fold). Fetal wounds showed a rapid but modest increase in collagen synthesis above an already high level of constitutive expression. In contrast, adult wounds showed a delayed but marked increase in collagen synthesis that reflected tremendous influx of new wound-specific fibroblasts and marked induction of collagen synthesis in native dermal fibroblasts. Scar-free repair in the fetus seems to involve relatively small injury-related changes to an already revved-up system. Wound-breaking strength seems to be similar between fetal and adult animal models; however fetal wounds regain tensile strength more rapidly than adult wounds [76, 77]. The mechanisms underlying this phenomenon are unknown but probably relate to differences in the type of collagen structure.
Fibromodulin
Fibromodulin is a member of the family of small interstitial leucine-rich proteoglycans (SLRP) that also include decorin. It is encoded by the gene FMOD [78]. Fibromodulin participates in the assembly of the collagen fibers of the extracellular matrix. It binds to the same site on the collagen type I molecule as lumican [79] and inhibits fibrillogenesis of collagen type I and collagen type III in vitro [80, 81]. It also regulates TGF-beta activities by sequestering TGF-beta into the extracellular matrix. Sequence variations in this gene may be associated with the pathogenesis of high myopia. Fibromodulin is found in the epidermis of human skin and is expressed by skin cells (keratinocytes) in culture. Fibromodulin knockout mice have very fragile skin [82], abnormal tails, and Achilles tendons and manifest several clinical features of Ehlers-Danlos syndrome [83]. The collagen fiber bundles in these tendons are scarcer and disorganized. The levels of lumican increased fourfold in the tail tendons of the FMOD-knockout mice. Liposomal-based delivery of the fibromodulin gene was investigated in a rat model with Achilles tendon injury. Encouraging results were observed that may provide new clinical therapeutic strategies [84]. In a recent study using loss- and gain-of-function rodent fetal wound models, fibromodulin was found to be essential for scarless wound healing. In particular, the loss of fibromodulin can eliminate the ability of early-gestation fetal rodents to heal without a scar. Administration of the protein alone was capable of restoring scarless healing in late-gestation rat fetal wounds, with dermal appendage restoration and organized collagen deposition that were virtually indistinguishable from those in controls with unwounded skin. High fibromodulin levels correlated with decreased transforming growth factor (TGF)-β1 expression and scarless repair, while low levels correlated with increased TGF-β1 expression and scar formation. Hence the FM-TGF-β1 nexus potentially plays a crucial role in fetal-type scarless skin repair [85].
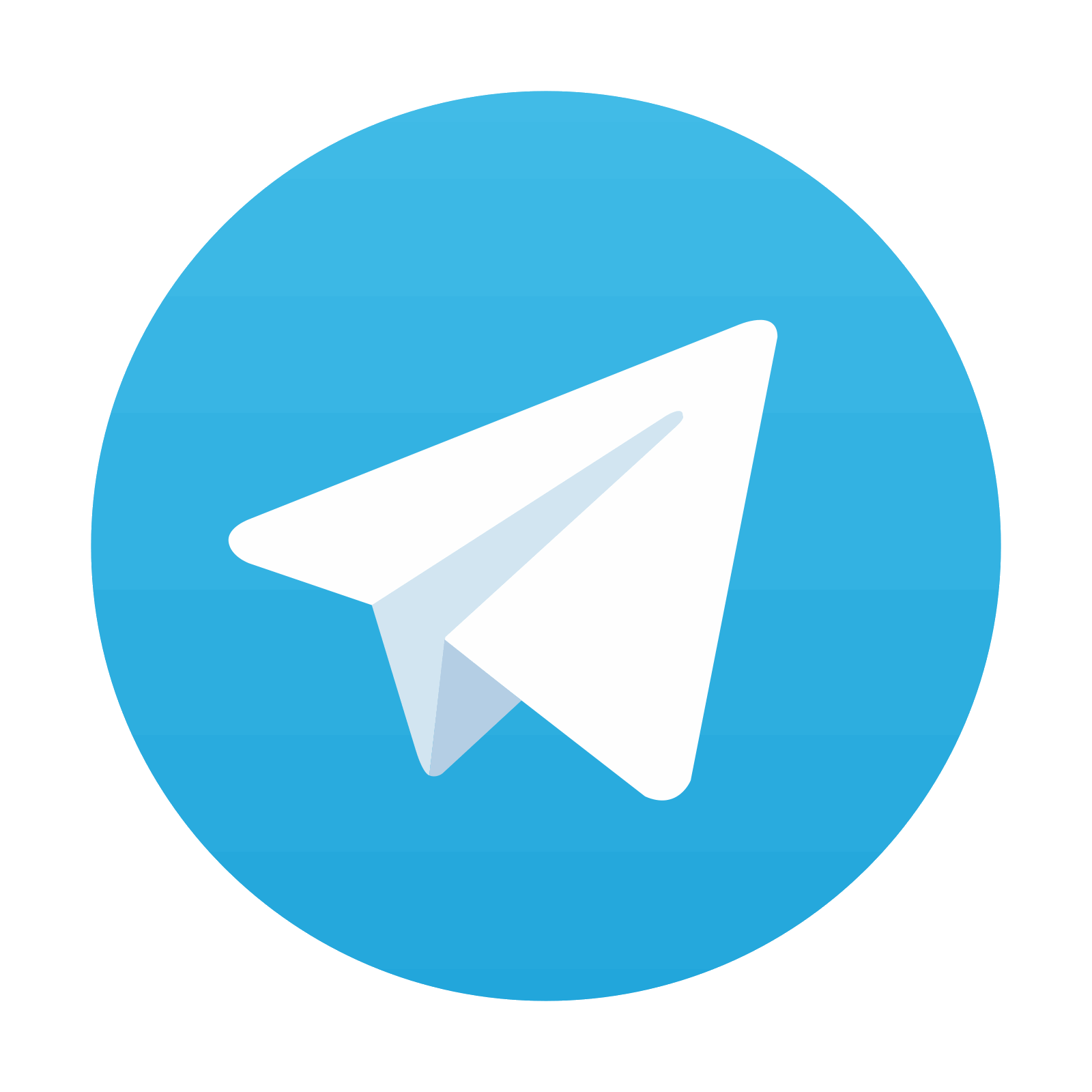
Stay updated, free articles. Join our Telegram channel
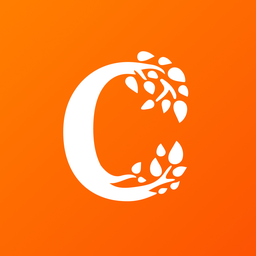
Full access? Get Clinical Tree
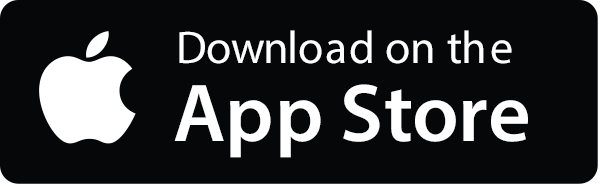
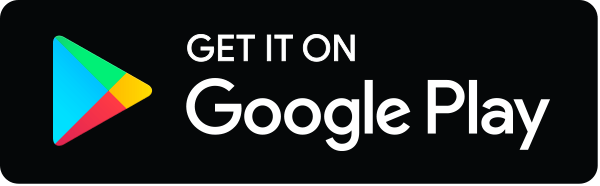