, Maneli Mozaffarieh1 and Hans Bebie2
(1)
Department of Ophthalmology, University of Basel, Basel, Switzerland
(2)
Institute for Theoretical Physics, University of Bern, Bern, Switzerland
Abstract
Ophthalmologists mainly use light for examinations and therapies, but not all tissues are accessible to light. A typical example is the contents of the orbit, behind and beside the eye. This is why ultrasound examinations also have an important place in our area of expertise. Ultrasound imaging relies on the detection of ultrasound reflected back from inhomogeneities. High frequencies are used to attain good resolution that can today approach fractions of a millimeter. Limits are set by the fact that higher frequencies are more strongly attenuated in water and tissue than lower frequencies.
Ophthalmologists mainly use light for examinations and therapies, but not all tissues are accessible to light. A typical example is the contents of the orbit, behind and beside the eye. This is why ultrasound examinations also have an important place in our area of expertise. Ultrasound imaging relies on the detection of ultrasound reflected back from inhomogeneities. High frequencies are used to attain good resolution that can today approach fractions of a millimeter. Limits are set by the fact that higher frequencies are more strongly attenuated in water and tissue than lower frequencies.
5.1 Sound and Ultrasound
Physically speaking, audible sound and ultrasound represent the same phenomenon, with the only difference being the frequency. As an example, we know about reflected sound in the audible range (Greek mythology gave it the name of the mountain nymph Echo); bats employ ultrasound echoes for their orientation. Sound and ultrasound spread out in gases and fluids by alternating compression and decompression phases. Here, the individual molecules vibrate longitudinally about their rest locations (Fig. 5.1). At boundary surfaces, the vibrations go over from one medium into another one.
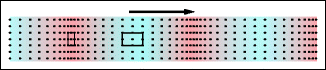
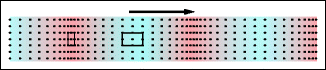
Fig. 5.1
Snapshot of sound vibrations. The compressed (red) and decompressed (blue) regions arise through the vibrations of the molecules about their resting positions. The dots indicate the positions of molecules
Beyond the audible range (16 Hz to 10–20 kHz, depending on age) is the ultrasound range, starting at frequencies above 20 kHz. The propagation velocity depends only very slightly on the frequency and is, thus, the same for audible sound and for ultrasound. It is highly dependent on the medium: 340 m/s in air, 1,480 m/s in water, and approximately 3,600 m/s in bones. In analogy to optics, with sound, one also encounters typical wave phenomena such as reflection, refraction, diffraction, scattering, absorption, and the Doppler effect. In ultrasound diagnostics, these all play a role in locating structures and movements. Thus, reflections are of particular importance.
Ultrasound is interesting for applications in ophthalmology because locations in the eye can be observed that are not accessible optically (Fig. 5.2). Locating a sound-reflecting object is possible only with a certain limited precision, on the order of the wavelength of the sound signal that is used. The wavelength of sound or ultrasound is given by the sound velocity divided by the frequency. Thus, the higher the frequency, the better the spatial resolution will be. For 10 MHz ultrasound, the wavelength in a watery medium is about 0.15 mm. The use of much higher frequencies – very attractive from the point of view of resolution – is limited to applications in the anterior chamber due to strongly increasing absorption (see Sect. 5.1.1).
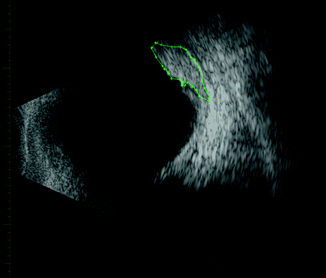
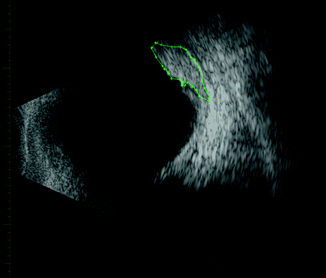
Fig. 5.2
Ultrasound image of a tumor in the eye (melanoma). Frequency 7.5 MHz (corresponding to a wavelength of 0.2 mm)
Bats and dolphins are among the best known animals that utilize ultrasound for perception in their environment (Fig. 5.3). Their analysis of the echo is so perfect that not only is an object located but it is also possible for them to recognize the shape of the object reflecting the ultrasound waves. Bats typically send out ultrasound pulses several times per second in a frequency domain of 40–140 kHz. Their ability to hunt insects even in total darkness was long a mystery. In 1795, the Italian researcher Spallanzani observed how bats, in spite of being blinded, could orient themselves perfectly, and in 1798, Ludwig Jurin, a physician in Geneva, saw that bats lost their orientation when their ears were plugged. It was only in 1938 that Donald Griffin and Robert Galambos – students at Harvard University at the time – were able to uncover the secret as they were able to register the bats’ sound signals electrically and, based on the frequencies, identify them as ultrasound.
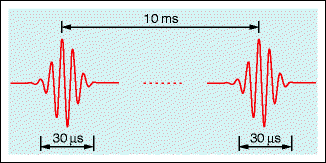
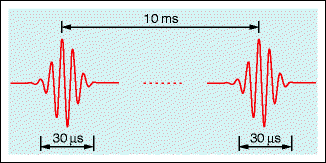
Fig. 5.3
Among other signals, dolphins send out sequences of ultrasound pulses to locate and scan objects based on their echoes. The figure shows two typical pulses from a series with regular intervals. The intervals between the pulses are long enough that an echo has time to return. Repetition frequency ≈ 102 Hz; ultrasound frequency ≈ 105 Hz. Number and pulse forms are just examples; depending on the species, they can vary a lot. In addition, dolphins in groups use lower frequencies to whistle to each other. The pulse-echo technique is also used in medicine
The first technical applications after 1920 were employed for locating icebergs and submarines as well as material testing. Austrian neurologist Karl Dussik was the first physician to use ultrasound for diagnostic purposes. He sent waves of 1.5 MHz through the head from one side and registered the local attenuations on the other side, producing a two-dimensional display (Fig. 5.4). His publications of 1942 and 1947 mark the beginning of ultrasound applications in medical diagnostics. Following a discussion of physical aspects, we shall treat applications in the eye.
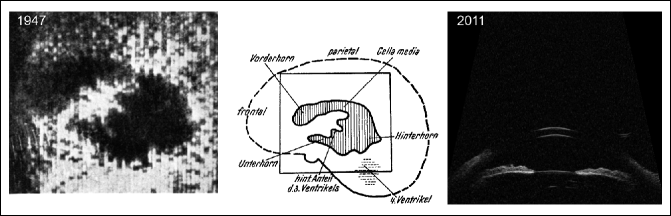
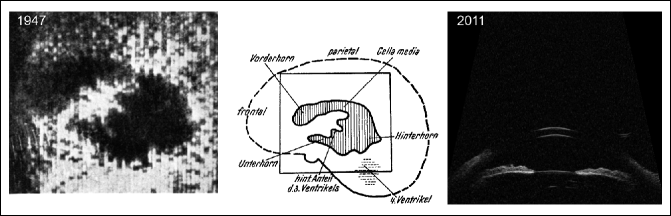
Fig. 5.4
Left: one of the first ultrasound images was taken of the human skull by Karl Dussik (1947). When passing through the ventricle, the beam is less attenuated. Middle: the explanation from the original publication. Right: modern ultrasound image with high resolution (biomicroscopic image of the anterior segment of the eye, 50 MHz)
5.1.1 Frequency, Wavelength, Resolution, Attenuation
Normally, ultrasound for diagnostic purposes is created by a longitudinally vibrating crystal (see Sect. 5.1.4). A sound emitter (as shown in Fig. 5.5) creates a beam that initially has a cylindrical shape and then begins gradually to widen. Imaging in the eye uses focused beams. Focal diameters down to an order of magnitude of one wavelength are possible.
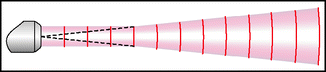
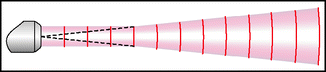
Fig. 5.5
Transducer with a plane exit surface. In the near field, the beam has a cylindrical shape and then begins gradually to widen
Today, technology permits a wide range of wavelengths (and thus frequencies) to be selected.1 The spatial resolution is limited to the order of one wavelength. High frequency means good resolution. The price paid for using a shorter wavelength is an increase in attenuation (Fig. 5.6). The decrease in sound intensity2 with increasing penetration depth is exponential. Note that the reflected wave on its way back to the receiver is subject to the same attenuation once again. The attenuation in water is much less than in air, while in biological tissues, it is intermediate.
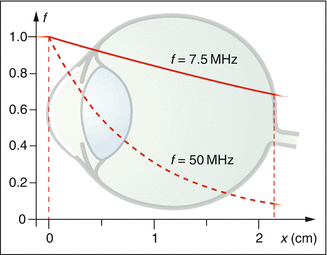
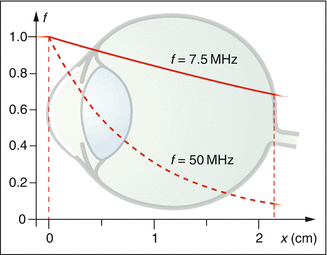
Fig. 5.6
Decrease in the intensity with increasing penetration depth. Higher-frequency ultrasound is more attenuated. Attenuation is exponential: if, at a certain frequency, the intensity decreases to half after a distance of 6 mm, it will again be halved along the next 6 mm, and so forth
A conventional ultrasound in ocular diagnostics of 10 MHz can be used up to a depth of 30–40 mm and produces a resolution on the order of 0.5 mm. In so-called ultrasound biomicroscopy, frequencies of 50 MHz or more are applied. Thus, a resolution down to approximately 50 μm is possible but at the cost of reduced working depth, which then amounts to only a few millimeters.
In a homogenous medium, the attenuation is mainly due to the absorption of sound energy and its conversion into heat. This process is energetically far more important than the relatively weak scatter due to small inhomogeneities such as blood, for example. While the heat can be therapeutically useful, only the backscattered ultrasound is diagnostically important.
5.1.2 Reflection, Refraction, Scattering, and Diffraction of Ultrasound
As with the interaction of light with matter, with ultrasound, the phenomena of reflection, refraction, scattering, and diffraction also occur and waves arise with new propagation directions. Of primary interest are the processes that send sound back into the sensor. These include reflection at a smooth interface between two media, reflection at an uneven or rough interface, or scattering at small particles and inhomogeneities (Fig. 5.7). Only the small portion of energy that returns to the sensor can be used diagnostically.
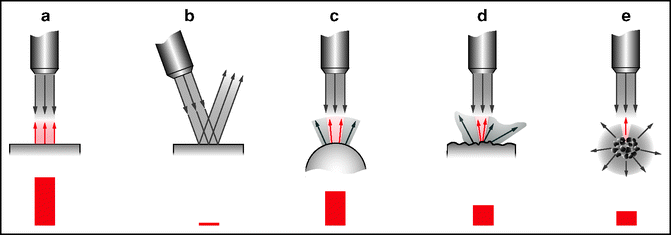
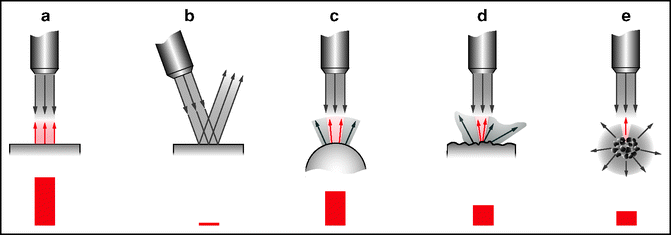
Fig. 5.7
Reflection of ultrasound. (a) Smooth interface between two media, perpendicular incidence. (b) Slanting incidence. (c) A curved interface. (d) Rough surface or interface. (e) Scattering at particles or inhomogeneities. The differing portions of energy that return to the sensor (built into the probe) are indicated by the red bars
Motionless inhomogeneities leave the frequency unaltered, while moving objects (such as erythrocytes in flowing blood) cause a Doppler shift in the frequency of the scattered sound (see Sect. 5.3).
For a reflection at a flat, smooth interface, the angle of incidence and reflection are the same (Fig. 5.7a and b). A slanting incident wave of sound may not return to the signal producer and is lost as an echo signal (if the sensor is built into the probe). When the reflecting surface is smooth but curved, the reflected wave is widened (Fig. 5.7c) and the amount of power that can return to the sensor is less than with a perpendicular incidence on a flat interface. In diffuse reflection at an uneven, rough interface, the sound is sent off in all directions (Fig. 5.7d) and the portion of the reflected power that arrives at the sensor is relatively small. Finally, particles or inhomogeneities present in a three-dimensional medium also create a diffuse reflection in all directions (Figs. 5.7e and 5.8). Even an individual cell represents a number of tiny reflectors. Small impediments are set to vibrate by the incident sound and, for their part, act as active sources of sound. Because the sound from these sources is sent out in all directions, the amount that returns to the sensor is small; the echo can, thus, be relatively weak. Note that not only the interfaces but also three-dimensional structures can act as sources of echoes.
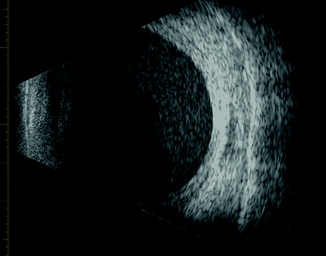
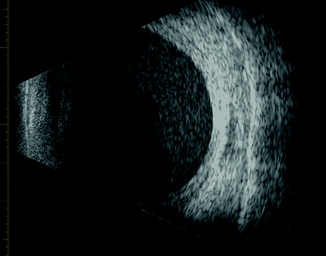
Fig. 5.8
B mode in an eye with vitreous hemorrhage. The local strength of the echo is coded as a gray value
Why does one have to put the sound probe either directly on the lid or introduce the sound into the eye via a fluid-filled funnel (Fig. 5.9)? In the transition of the ultrasound from the air into the eye, almost all the energy would be reflected by the cornea for a purely physical reason. In a transition from air to water or vice versa, the differing densities are mainly responsible for the high degree of reflection (a digression with a more precise explanation can be found in Sect. 5.1.3).
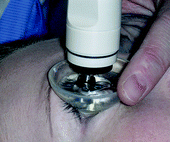
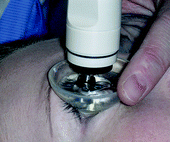
Fig. 5.9
Funnel in place
At the interface between two media of differing sound velocities (Fig. 5.10), the sound wave is refracted; that is, it continues in an altered direction. The refracted beam is closer to the surface normal when the new medium has a lower sound velocity; otherwise, it is positioned away from the vertical. This means that a certain distortion cannot be avoided. For applications in the eye, this is of secondary importance. In daily experience, for an attentive observer, a refraction of audible sound occurs in situations such as when a train passes behind a flat hill. The train will not be visible but can be heard with greatly differing volume, depending on whether the temperature gradient in the air above the hill points in an upward or downward direction – which depends on the weather conditions (Fig. 5.11). Acoustic lenses that can focus sound represent an application of refraction, analogous to optical lenses.
< div class='tao-gold-member'>
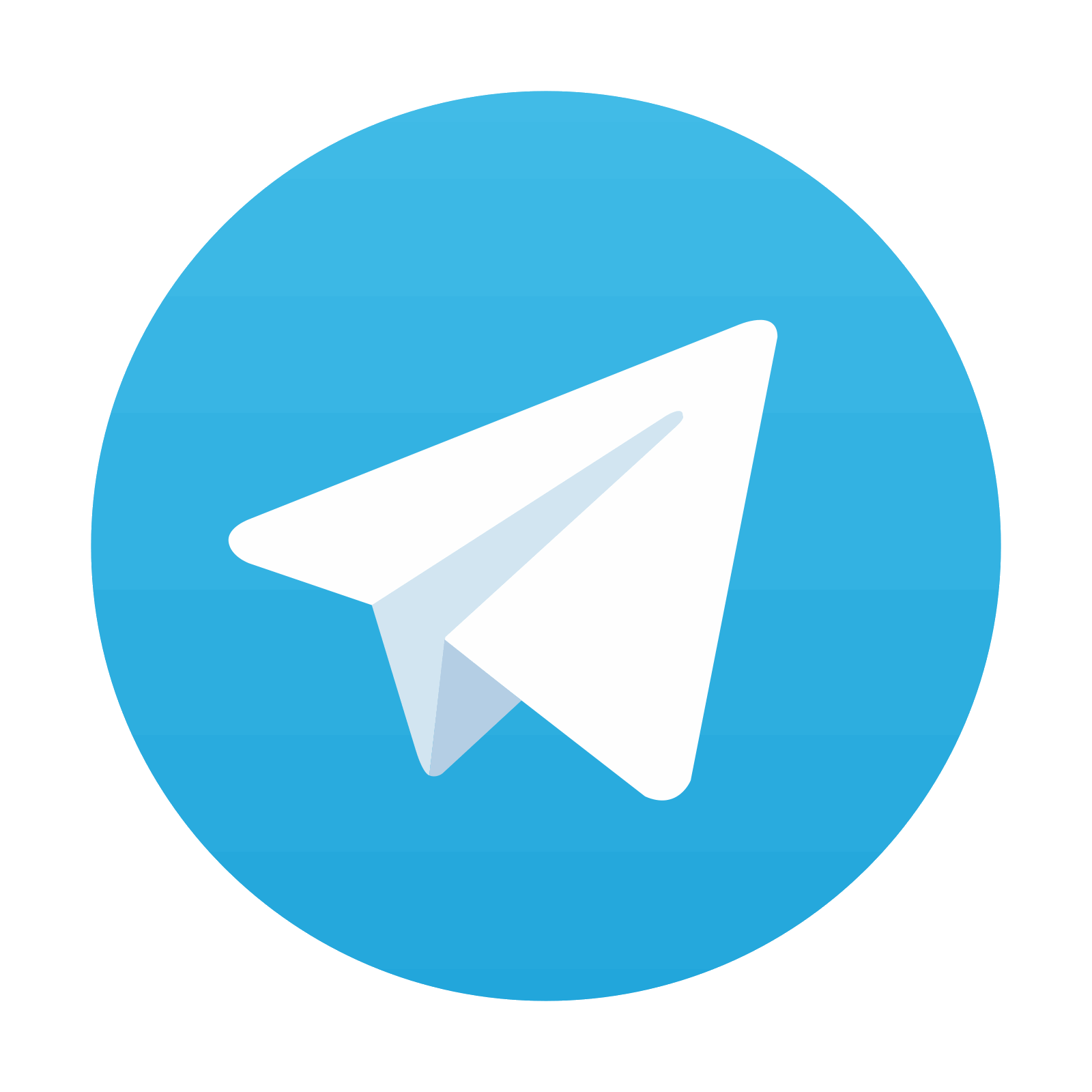
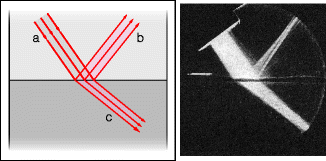
Only gold members can continue reading. Log In or Register a > to continue
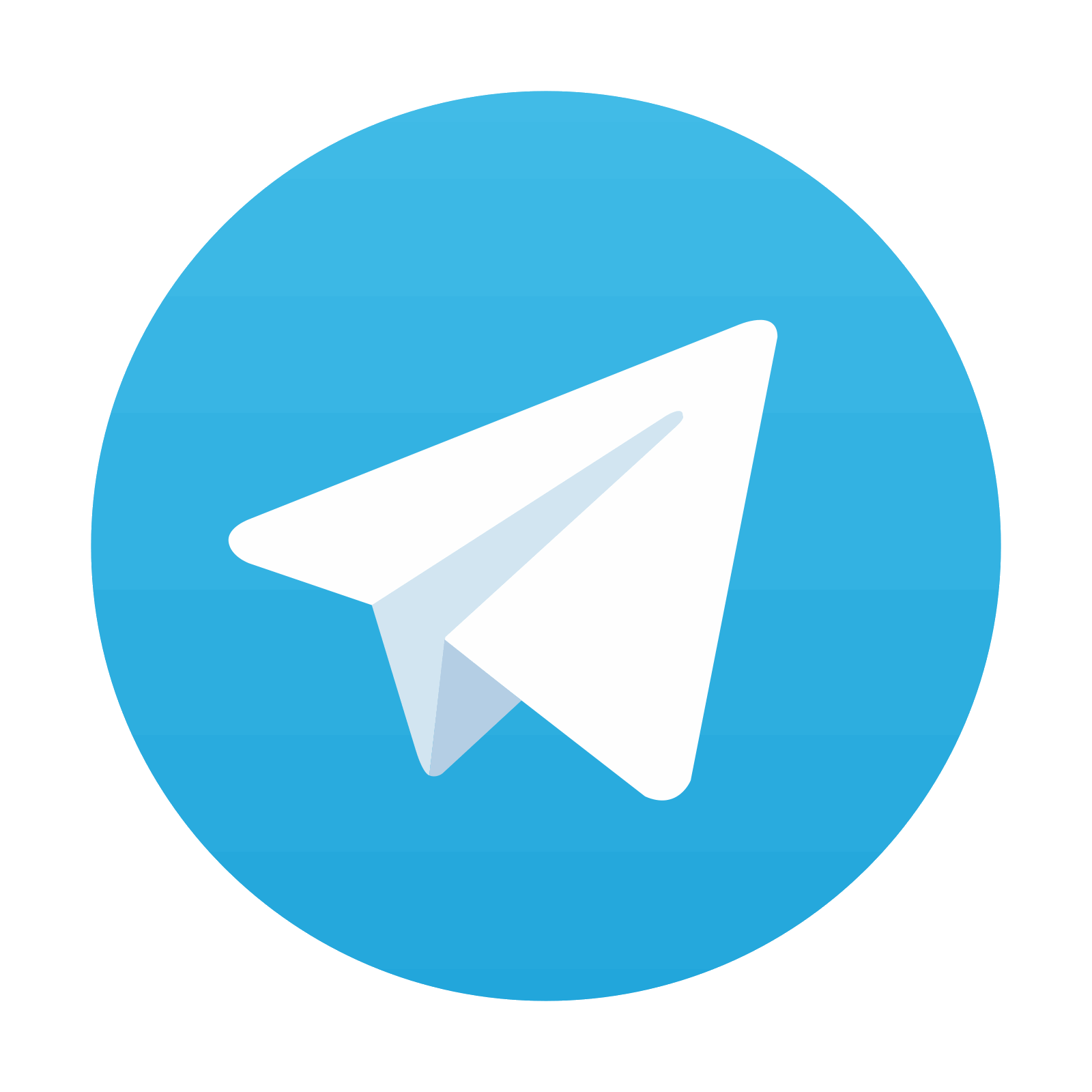
Stay updated, free articles. Join our Telegram channel
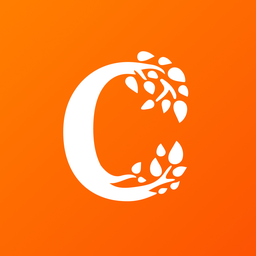
Full access? Get Clinical Tree
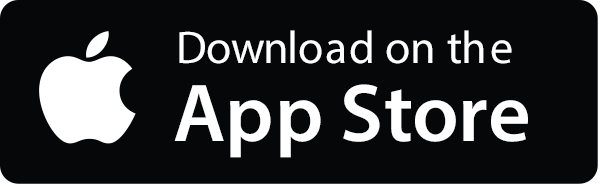
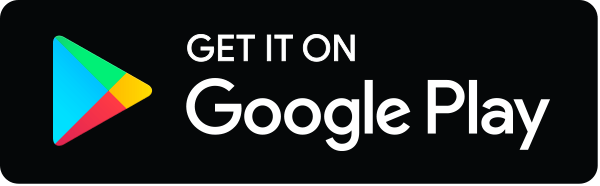
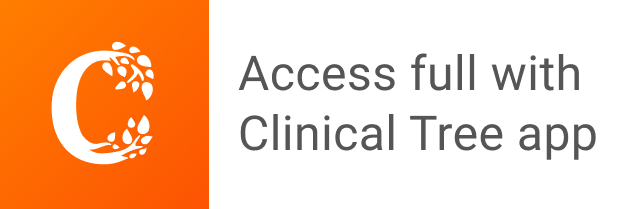