Agent
Starting dose
Route
Use
Calcium gluconate
1–2 g
Intravenous
Acute hypoparathyroidism
Calcium salts
500–1000 mg of elemental calcium three times daily
Oral
Chronic hypoparathyroidism
Vitamin D metabolites
See table 35.2
–
–
Hydrochlorothiazide
25–100 mg daily
Oral
Hypercalciuria
Chlorthalidone
25–100 mg daily
Oral
Hypercalciuria
PTH 1-34
0.5–0.7 mcg/kg dailya
Subcutaneous
Chronic hypoparathyroidism
PTH 1-84
50–100 mcg daily
Subcutaneous
Chronic hypoparathyroidism
Chronic Management
Calcium
Patients with asymptomatic or minimally symptomatic hypocalcemia can be managed in the outpatient setting. Calcium carbonate and calcium citrate are widely accepted forms of calcium supplementation (Table 35.1). In general, studies on the bioavailability of calcium carbonate and calcium citrate demonstrate conflicting results depending on the population studied and the methodology [10–12]. Premenopausal women treated with calcium carbonate exhibited improved calcium bioavailability measured by serum total and ionized calcium and PTH compared to treatment with calcium citrate [12]. Studies in postmenopausal women have demonstrated no difference or superior bioavailability of calcium citrate [10, 11, 13]. In addition to menopausal status, gastric acid secretion may account for some of these differences. In the presence of achlorhydria or diminished gastric acid output, as can be seen with proton-pump inhibitors and H2 blockers, calcium citrate provides superior bioavailability because calcium carbonate requires an acidic environment for absorption [14]. Calcium carbonate and calcium citrate contain 40 and 21 % of elemental calcium, respectively. In general, 1–2 g of elemental calcium is administered three times a day.
Vitamin D Metabolites and Analogs
In addition to calcium, treatment with vitamin D metabolites or analogs is critical in the management of hypoparathyroidism by improving intestinal calcium absorption and potentially by promoting bone remodeling ([15, 16]; reviewed in [17]). Vitamin D is available in several forms, which differ in onset of action, half-life, and cost (Table 35.2). Cholecalciferol (viamin D3), which is cutaneously formed or obtained in the diet, has a half-life of approximately 20 h. Ergocalciferol (vitamin D2), which is manufactured from yeast, has been used to treat severe vitamin D deficiency because historically it has been available in higher doses than cholecalciferol. However, several studies suggest that ergocalciferol is 3 times less potent, has a slower onset of action (3 days vs. 14 days) and has a shorter duration of action than cholecalciferol [18–21]. Calcidiol (25-hydroxyvitamin D3), which is not commonly used for pharmacologic replacement, is produced by hydroxylation of cholecalciferol in the liver and has low biologic activity, but is the major metabolite in the bloodstream. Calcidiol is further hydroxylated in a PTH-dependent manner by renal 1α-hydroxylase in the kidney resulting in formation of the most active metabolite, calcitriol. Because of a rapid onset of action and no requirement for PTH-dependent conversion, calcitriol results in improvement of hypocalcemia within hours. The potency of calcitriol can result in hypercalcemia, however, this is quickly reversed upon discontinuation of calcitriol due to the short half-life of 4–6 h. Alfacalcidol (1-hydroxyvitamin D3) and doxercalciferol (1-hydroxyvitamin D2) are synthetic analogs that can be converted to calcitriol in the liver. Like calcitriol, alfacalcidol and doxercalciferol (1) do not require PTH-mediated activation, (2) have a rapid onset of action and short half-life, and (3) can induce hypercalcemia [22–26]. Doxercalciferol appears to be less prone to hypercalcemic episodes than calcitriol and alfacalcidol; however, these studies were performed in patients with secondary hyperparathyroidism and animals models, so their applicability to hypoparathyroidism is limited [26–28]. Prior to the availability of metabolically active forms of vitamin D, high doses of cholecalciferol or ergocalciferol were used to treat hypoparathyroidism with some success, perhaps due to (1) low affinity binding of cholecalciferol or ergocalciferol to the vitamin D receptor when supplemented at high doses and (2) limited conversion of cholecalciferol or ergocalciferol to calcitriol despite absence of PTH. At present, active metabolites of vitamin D are primarily used in the treatment of hypoparathyroidism. Cholecalciferol or ergocalciferol supplementation in addition to active metabolites of vitamin D may be beneficial because of a longer half-life and limited peripheral tissue-mediated conversion to calcitriol; however, further studies are needed to substantiate this possibility [29, 30].
Table 35.2
Vitamin D metabolites
Vitamin D preparation | Starting dose | Onset of action | Plasma half-life | Cost |
---|---|---|---|---|
Cholecalciferol | 25,000 IU/d | 8–72 h | 14–25 h | $ |
Ergocalciferol | 50,000 IU/d | 10–24 h | 19–48 h | $ |
Calcidiol | 0.25 mcg/d | 12 h | 16–19 days | – |
Alfacalcidol | 0.25 mcg/d | 6 h | 3 h | $$ |
Doxercalciferol | 1 mcg/d | Unknown | 32–37 h | $$$$$ |
Calcitriol | 0.25 mcg/d | 2–6 h | 3–8 h | $$ |
Management of Calcium and Vitamin D-Related Complications
Most complications that occur due to treatment of hypoparathyroidism are related to vitamin D toxicity, which can result in hyperphosphatemia, hypercalcemia, and hypercalciuria. Vitamin D supplementation enhances intestinal absorption of calcium and phosphorus. If hyperphosphatemia occurs, initial treatment is to reduce dietary intake. Phosphate binders are reserved for severe situations. Hypercalcemia may be short-lived or prolonged depending on the form of vitamin D replacement. Hypercalciuria can occur in the absence of hypercalcemia due to poor renal calcium resorption [25]. Thiazide diuretics are used in prevention and treatment of hypercalcemia and hypercalciuria.
Thiazide Diuretics
Thiazide diuretics reduce urinary calcium excretion by increasing distal renal tubular calcium reabsorption (Table 35.1). Although some studies on surgical hypoparathyroidism suggested that this reduction of urine calcium is PTH-dependent [31–33], other studies indicate that decreased urinary calcium does not require presence of PTH [34]. Larger studies delineating the cause of hypoparathyroidism, the quantity of PTH, and the effect of thiazide diuretics are warranted. Until such studies are available, a trial of thiazide diuretics is indicated in hypoparathyroid patients with hypercalciuria. Thiazide diuretics are most likely to be beneficial in hypoparathyroid patients with activating CaSR mutations where treatment of hypocalcemia frequently results in hypercalciuria [35, 36]. In addition to serum calcium, potassium and magnesium levels should be monitored and supplemented if necessary. Treatment with a thiazide diuretic should be discontinued if it fails to reduce urine calcium or results in hypotension.
Calcilytics
Activation of the parathyroid CaSR results in increased intracellular Ca2+ and decreased PTH secretion. CaSR is expressed in parathyroid chief cells and in all nephron segments in the kidney. Activating CaSR mutations cause inappropriately low PTH levels in the setting of hypocalcemia. Although activation of renal CaSR primarily increases urinary calcium excretion, it also inhibits resorption of potassium, sodium, and water (reviewed by [37]). Calcimimetics potentiate the CaSR and inhibit PTH secretion and are useful in treatment of hyperparathyroidism whereas calcilytics inhibit the CaSR and can treat certain forms of hypoparathyroidism [38]. One of the early calcilytics, NPS 2143, reduced intracellular calcium in human embryonic kidney cells, stimulated PTH secretion from bovine parathyroid cells in vitro, and increased serum calcium in parathyroidectomized rats [38–41]. Because of promiscuous action against norepinephrine, dopamine, and serotonin monoamine transporters by NPS-2143, three other calcilytics, ronacaleret (SB-751689), NPSP795, and JTT-305/MK-5442, with a more desirable selectivity profile were developed. Ronacaleret administration in a randomized placebo-controlled trial of 569 postmenopausal women with osteoporosis resulted in increased serum calcium, PTH, and lumbar spine bone mineral density [42]. However, decreases in bone mineral density of the total hip, femoral neck, and trochanter were seen. The effects of ronacaleret in hypoparathyroidism have yet to be studied. Similarly, treatment with JTT-305/MK-5442 increased serum calcium, PTH, and bone formation markers in a randomized placebo-controlled trial on 383 postmenopausal women with osteoporosis [43]. NPSP795 is currently in Phase II clinical trials for treatment of hypoparathyroidism secondary to activating CaSR mutations. The efficacy of these medications in patients with hypoparathyroidism due to activating CaSR mutations will be critical because of the complications caused by calcium and vitamin D replacement, as well as the ineffectiveness of PTH replacement.
Synthetic PTH
Treatment of hypoparathyroidism with the standard agents of calcium and vitamin D leaves patients vulnerable to psychiatric illnesses and increased morbidity from infections while exposing patients to the risks of renal and vascular disease. A retrospective U.S. study of patients with hypoparathyroidism indicated increased risk of chronic renal insufficiency and hospital admission related to hypoparathyroidism [44]. Nationwide Danish case-control studies in patients with postsurgical hypoparathyroidism demonstrated a fivefold increased risk of renal stones and renal insufficiency, twofold increased incidence of depression or bipolar disease, and increased risk of hospitalizations due to infection or seizure [45, 46]. In nonsurgical hypoparathyroidism, there was an increased risk of renal insufficiency, cardiac ischemia and arrhythmia, stroke, depression, and hospitalization due to infection in a similarly structured Danish case-control study [47]. One small case-control study demonstrated that intimal thickening of arteries was elevated in patients with hypoparathyroidism suggesting intimal thickening as a mechanism for risk of cardiovascular disease [48]. Together, these data imply that treatment of hypoparathyroidism with calcium and vitamin D cannot adequately replace PTH. Thus, interest in PTH replacement therapy has increased. Currently available pharmacologic replacement therapies include recombinant human PTH 1-34 and PTH 1-84 (Table 35.1, Fig. 35.1). Both proteins must be administered subcutaneously due to poor oral bioavailability.


Fig. 35.1
Recombinant human PTH. PTH 1-34 and PTH 1-84 both contain two protein kinase C (PKC) domains and an adenylate cyclase (AC) domain, which are located in the N-terminus. PKC and AC activate the PTH receptor on chondrocytes, osteoblasts, and kidney cells. Only PTH 1-84 retains the C-terminus, which may stimulate bone resorption by osteoclasts through an alternative receptor
Bovine PTH
PTH 1-34
Initial studies on PTH replacement were performed using the biologically active N-terminus of the PTH molecule (Fig. 35.1). One case study demonstrated increased serum calcium after treatment with PTH 1-38 [51]. Subsequent studies used PTH 1-34. A small 5-month pilot study randomized ten patients with hypoparathyroidism to daily subcutaneous PTH 1-34 injections or calcitriol. Those patients treated with PTH 1-34 demonstrated normal serum calcium and decreased urinary calcium suggesting superiority compared to calcitriol [52]. These results were confirmed in a 3-year randomized control trial of 27 patients with hypoparathyroidism [53]. Urine calcium was reduced with PTH 1-34 treatment, but this was not statistically significant, likely due to small sample size. This study also demonstrated no differences in bone mineral density, although markers of bone mineral turnover were increased with PTH 1-34 treatment. Additional studies by the same group demonstrated that titration of PTH 1-34 dose to twice daily dosing or continuous dosing via pump resulted in uniform serum calcium and further reduction of urinary calcium compared to daily dosing regimens [54, 55].
Unfortunately, long-term supraphysiological doses of PTH 1-34 given to rats with normal functioning parathyroid glands led to osteosarcomas [56]. This risk was not recapitulated in postmenopausal women who were given PTH 1-34 for 2 years for the treatment of osteoporosis [57]. Furthermore, a trial of 12 pediatric patients with hypoparathyroidism treated with twice-a-day PTH 1-34 for 3 years vs. calcitriol, which was initiated prior to the toxicology studies in rats, demonstrated no differences in serum or urine calcium, bone mineral density, growth, or adverse events including development of osteosarcoma during the 3-year follow-up [58].
Despite these findings, enthusiasm for long-term use of PTH 1-34 for hypoparathyroidism, especially in pediatric populations, has waned. Currently, use is restricted to treatment of osteoporosis in adults for no more than 2 years. Surveillance studies to identify the risk of osteosarcoma with long-term (15 years) use in adults are ongoing, and interim results demonstrate no risk of osteosarcoma [59]. The final report is expected to be available in 2019.
PTH 1-84
A case series of 30 hypoparathyroid patients using PTH 1-84 demonstrated decreased requirements for calcium and vitamin D metabolites [60]. This study showed no decrease in urinary calcium but did identify an increase in bone mineral density at the lumbar spine. These findings were confirmed in a randomized double-blind trial of 62 female patients with postsurgical hypoparathyroidism in which PTH 1-84 or placebo was added to a regimen of calcium and a vitamin D metabolite [61]. As expected, use of PTH 1-84 significantly reduced calcium and vitamin D metabolite requirements. Treatment with PTH 1-84 resulted in more episodes of hypercalcemia than placebo; however, this is likely due to lack of supplement titration until patients developed hypercalcemia. Although there was an initial increase in urine calcium with PTH 1-84 compared to placebo, no difference was noted for the remainder of the study. Furthermore, this study demonstrated a decrease in bone mineral density in the whole body, spine, and femoral neck in patients receiving PTH 1-84. A larger Phase 3 randomized placebo-controlled double-blind trial of 134 hypoparathyroid patients confirmed reduction of calcium and vitamin D supplementation with PTH 1-84 treatment and showed similar adverse events with PTH 1-84 compared to placebo [62]. Both placebo and PTH 1-84 treated groups demonstrated a decreased in urine calcium, but, surprisingly, the decrease from baseline in the placebo group was significantly greater than the PTH 1-84 group. Although these studies suggest limited renal protection by PTH 1-84, a recent open label study of 7 patients who received a single dose of PTH 1-84 demonstrated 12–26 % reduction of urine calcium and increased creatinine clearance compared to baseline parameters while on calcitriol [63]. Further studies are necessary to determine if PTH 1-84 will reduce the risk of nephrolithiasis and renal insufficiency. One 5-year open label study demonstrated improved quality of life with PTH 1-84; however, a subsequent 6-month randomized controlled trial demonstrated no improvement in quality of life [64, 65]. Again, additional long-term studies are warranted to determine if PTH 1-84 confers protection against psychiatric disease. Similarly, studies will be necessary to identify cardiovascular protection afforded by PTH 1-84. In these future studies, it will be important to analyze outcomes after patients are classified by surgical and nonsurgical hypoparathyroidism, given that risks may vary between these patient populations [45–48].
Initially, the N-terminus of the PTH protein was considered to be the biologically active fragment; however, mounting evidence suggests that the C-terminus also contributes to PTH function. Recombinant human PTH 1-84 and PTH 1-34 demonstrate slight differences in pharmacokinetics—half-life (1 vs. 1.5 h), peak post injection (0.5 vs. 1-3 h), calcium peak (4-6 vs. 6-8 h), and return to baseline calcium (16-24 vs. 24 h) [66–68]. Because of these structural and pharmacokinetic differences between PTH 1-34 and PTH 1-84, differences in treatment of hypoparathyroidism may be anticipated. A meta-analysis, which demonstrated 64 % risk reduction of vertebral fracture and increased spine bone mineral density in osteoporotic patients with use of either PTH 1-34 or 1-84, was unable to identify superiority of either hormone type [69]. To date, however, no comparative clinical trials have been performed.
Tissue and Cellular Transplants
The ideal treatment for hypoparathyroidism in most patients is replacement of functional, calcium-responsive parathyroid tissue. Although PTH hormone replacement therapy may provide some benefits over treatment with calcium and vitamin D, dosing regimens are still not physiologic. Several clinical and experimental models for transplantation of parathyroid tissue exist to prevent and treat hypoparathyroidism. Autotransplantation is performed in patients routinely, whereas allotransplantation is less common because of potential need for immunosuppression. Stem cell-derived parathyroid cells may offer restoration of physiologic parathyroid function in the future.
Autotransplant With and Without Cryopreservation
Hypoparathyroidism is most commonly secondary to neck surgery. Devascularization of the parathyroid glands can be intentional or unintentional. In the event of intentional devascularization or unintentional devascularization of the parathyroids identified intraoperatively, the parathyroid gland can be autotransplanted. In this scenario, the parathyroid gland is inspected for abnormality then placed in iced saline until the end of the operation. The gland is cut using fine scissors to create a suspension. The suspension is then implanted into a pocket created in the sternocleidomastoid muscle . An early case series consisting primarily of patients with secondary hyperparathyroidism demonstrated graft survival in 93 % of the 29 patients receiving autotransplants [70]. A later case series, in which autotransplantation of 1-2 parathyroid glands was routinely performed in conjunction with in situ preservation of remaining parathyroids, demonstrated normal long-term parathyroid function in 99 % of patients [71]. Function can reappear weeks to months after autotransplant. In general success rates vary from 85 to 99 % [72].
In addition to immediate autotransplantation, parathyroid tissue can be cryopreserved for transplantation at a later date. Cryopreservation can be considered in patients with hyperparathyroidism due to multigland disease where extent of resection may not be clear or likelihood of reoperation is high as with secondary and tertiary hyperparathyroidism (reviewed in [73]). In addition, extended dissection, repeat surgery, and thyroid malignancy were risk factors for postoperative hypoparathyroidism [74, 75]. Thus, cryopreservation in conjunction with autotransplantation can be considered in these situations. Cryopreservation is performed by dissecting parathyroid tissue into 1 × 1 × 1 mm fragments followed by placement in iced saline. After transport, the supernatant is decanted and the tissue is resuspended in freeze media typically consisting of 80 % RPMI 1640, 10 % fetal bovine serum, and 10 % dimethyl sulfoxide [76]. Reimplantation is performed in the nondominant forearm so that graft function can be determined by comparing blood from antecubital veins of the grafted and nongrafted arms. Cryopreservation retains function in less than 60 % of patients whereas immediate autotransplant retains function in over 90 % of patients [77–79]. Thus, autotransplant should be performed in place of or in conjunction with cryopreservation when feasible.
Allogeneic Transplants
The first successful allotransplantation of parathyroid tissue was performed in a patient who underwent subtotal parathyroidectomy for secondary hyperparathyroidism [80]. After renal transplant, she developed hypoparathyroidism, which was corrected with parathyroid allotransplant from another patient undergoing parathyroidectomy for secondary hyperparathyroidism. Additional studies confirmed the feasibility of parathyroid allotransplantation in the setting of immunosuppression [81–85]. However, for the majority of hypoparathyroid patients, the risk of immunosuppression outweighs the risk of parathyroid allograft. Thus, several models have been generated to reduce the immunogenicity of parathyroid allografts. Parathyroid tissue cultured in vitro and depleted of antigen presenting cells was used for allotransplantation and resulted in tissue survival for up to 18 months [86–89]. Nude mice have been used as an “interim host system” to condition human parathyroid tissue prior to allotransplantation, resulting in allograft function for up to 15 months post transplant in 2 patients [90]. More recent studies have adopted the technique of microencapsulation used in pancreatic islet cell transplantation [91–95]. Two patients receiving microencapsulated transplants demonstrated graft function 3 months after allotransplantation [96]. Another case report using microencapsulation demonstrated over 20 months of allograft function [97]. Translating the technique of microencapsulation from animal models to human allograft transplantation has met challenges. However, new approaches incorporating further modifications to the microcapsule generation are promising. A clinical trial, which is currently underway, will provide additional details regarding long-term viability of parathyroid allografts.
Stem Cell-Derived Therapy
Replacement of functional parathyroid tissue would provide the most physiologic treatment for hypoparathyroidism. Recent advances in allograft transplantation provide one avenue for cure; however, tissue sources for allogeneic transplantation may be pathologic or limited in availability. Developing a source of autologous PTH-producing cells from easily accessible tissue can obviate the constraints of contemporary treatment for hypoparathyroidism. Parathyroid organogenesis is carefully orchestrated by the actions of a network of transcription factors and signaling molecules that act on the pharyngeal endoderm and surrounding mesenchyme. A combination of human disorders associated with the parathyroid glands as well as murine experimental models have led to a greater understanding of this process. Several transcription factors critical to parathyroid development, such as Hoxa3, Pbx1, Pax1, Pax3, Pax9, Eya1, Six1, Tbx1, Gcmb (Gcm2 in mice), Aire1, and Gata3, have been identified [98–119]. Pharyngeal endoderm expresses Tbx1, Pax1, Pax9, Six1, Eya1 as well as Hoxa3 in the caudal region of the second pouch. These factors are important in specification of the third pharyngeal pouch and its derivatives including the parathyroid. Gata3 is required for expression of Gcm2, which is required for and specific to parathyroid organogenesis. Furthermore, many of these transcription factors can be used as markers of progenitor cell specification to the parathyroid lineage prior to differentiation into PTH-producing cells.
To date, parathyroid-like cells have been generated from embryonic stem cells (ESCs) as well as thymocytes, which share developmental origin with the parathyroid glands ([120]; reviewed in [121–124]). The Bingham protocol differentiates ESCs into definitive endoderm and then into PTH-producing cells using Activin A and increasing concentrations of fetal bovine serum for 5 days followed by removal of Activin A [120]. This protocol results in expression of Eya1, Six1, Pax1, GCM2, CaSR, and PTH [120]. Addition of sonic hedgehog appeared to increase CaSR and PTH mRNA expression, but did not increase PTH secretion [123]. A more complicated regimen of sequential treatment with BMP4/ROCK inhibitor, Activin A/BMP4/bFGF, NOGGIN/TGF-β pathway inhibitor, WNT3a/KGF/FGF10, BMP4/EGF, and FGF8 also resulted in GCM2 mRNA expression [122]. Studies have shown that human thymocytes express low levels of GCM2, CaSR, and PTH mRNA [124]; however, this may be due to the presence of low numbers of parathyroid cells retained in the thymus [125]. Separation of thymic epithelial cells and treatment with the Bingham protocol resulted in increased EYA1, GCM2, PAX1, CaSR, and PTH mRNA expression [124]. Furthermore, long-term culture of these cells resulted in calcium-responsive secretion of PTH. These transdifferentiated thymocytes produced supraphysiologic levels of PTH. Although transplantation into immunocompromised Rag2−/− mice did not result in tumorigenesis, hypercalcemia as a result of hyperparathyroidism was not assessed [124]. These studies hold great promise for parathyroid organogenesis and treatment of hypoparathyroidism; however, future research is still necessary to demonstrate function in parathyroidectomized murine models.
Treatment of Hypoparathyroidism During Pregnancy
Maintenance of calcium homeostasis is essential for normal fetal development. Hypocalcemia attributable to undertreatment of hypoparathyroidism during pregnancy can result in fetal hyperparathyroidism, bone malformation, and death [126, 127]. Overtreatment resulting in maternal hypercalcemia can cause fetal parathyroid hypoplasia and miscarriage. During pregnancy, normal maternal calcium levels are maintained despite calcium mobilization to the fetal skeleton due to elevated levels of vitamin D metabolites and PTH related protein [128, 129]. Calcitriol requirements may be reduced or increased in the pregnant hypoparathyroid patient [126, 130–136]; however, calcitriol requirements generally decrease during lactation [130–132, 134, 137]. Historically, calcitriol was withheld during pregnancy due to concern for teratogenicity; however, recent studies have suggested that this is unlikely [126, 138]. Because of fluctuating calcitriol requirements, serum calcium levels should be assessed frequently during pregnancy and lactation. Generally, calcitriol requirements return to baseline after cessation of lactation.
Summary
Acute and chronic hypoparathyroidism can result in cardiac, renal, and psychiatric disease. Acute treatment consists of intravenous calcium gluconate . The mainstay of chronic treatment consists of calcium and calcitriol. Thiazide diuretics can be used to mitigate calciuria and nephrolithiasis. Calcilytics, which are not yet widely available for treatment of hypoparathyroidism, offer the potential for improved calcium homeostasis and fewer complications, especially to patients with activating CaSR mutations. Hormone replacement therapies such as PTH 1-34 and PTH 1-84 may offer advantages over calcium and calcitriol; however, further studies are required to characterize potential renal protection by reduction of hypercalciuria. Autologous parathyroid transplant is frequently used when risk of hypoparathyroidism is deemed to be high preoperatively or intraoperatively. In general, immediate autotransplant is more successful than transplant after cryopreservation. Conversely, allogeneic parathyroid transplants have not shown long-term success, except in the setting of immunosuppression. Future studies should focus on generation of functional parathyroid cells from autologous sources such as pluripotent stem cells or thymus. Significant work remains to demonstrate that these cellular therapies are functional in vivo, and do not result in hyperparathyroidism. However, parathyroid organogenesis provides the possibility of freedom from complications and permanent cure.
Best Practices:
N/A
Expert Opinion
Treatment of hypoparathyroidism is an infrequent but potentially intense endeavor. It requires the providers attention to detail and a high level of patient education and cooperation. Advances in hormone replacement therapy, auto transplantation, and the possibility of stem cell therapy offer hope to this population of patients.
References
1.
Burge MR, Zeise TM, Johnsen MW, Conway MJ, Qualls CR. Risks of complication following thyroidectomy. J Gen Intern Med. 1998;13:24–31. Clinical Investigation; Level 2; Grade B.PubMedPubMedCentral
2.
Falk SA, Birken EA, Baran DT. Temporary postthyroidectomy hypocalcemia. Arch Otolaryngol Head Neck Surg. 1988;114:168–74. Clinical Investigation; Level 4.PubMed
3.
Percival RC, Hargreaves AW, Kanis JA. The mechanism of hypocalcaemia following thyroidectomy. Acta Endocrinol (Copenh). 1985;109:220–6. Clinical Investigation; Level 2b.
4.
Rosato L, Avenia N, Bernante P, De Palma M, Gulino G, Nasi PG, Pelizzo MR, Pezzullo L. Complications of thyroid surgery: analysis of a multicentric study on 14,934 patients operated on in Italy over 5 years. World J Surg. 2004;28:271–6. Clinical Investigation; Level 2; Grade B.PubMed
5.
See AC, Soo KC. Hypocalcaemia following thyroidectomy for thyrotoxicosis. Br J Surg. 1997;84:95–7. Clinical Investigation; Level 4.PubMed
6.
Hurley K, Baggs D. Hypocalcemic cardiac failure in the emergency department. J Emerg Med. 2005;28:155–9. Clinical Investigation; Level 5.PubMed
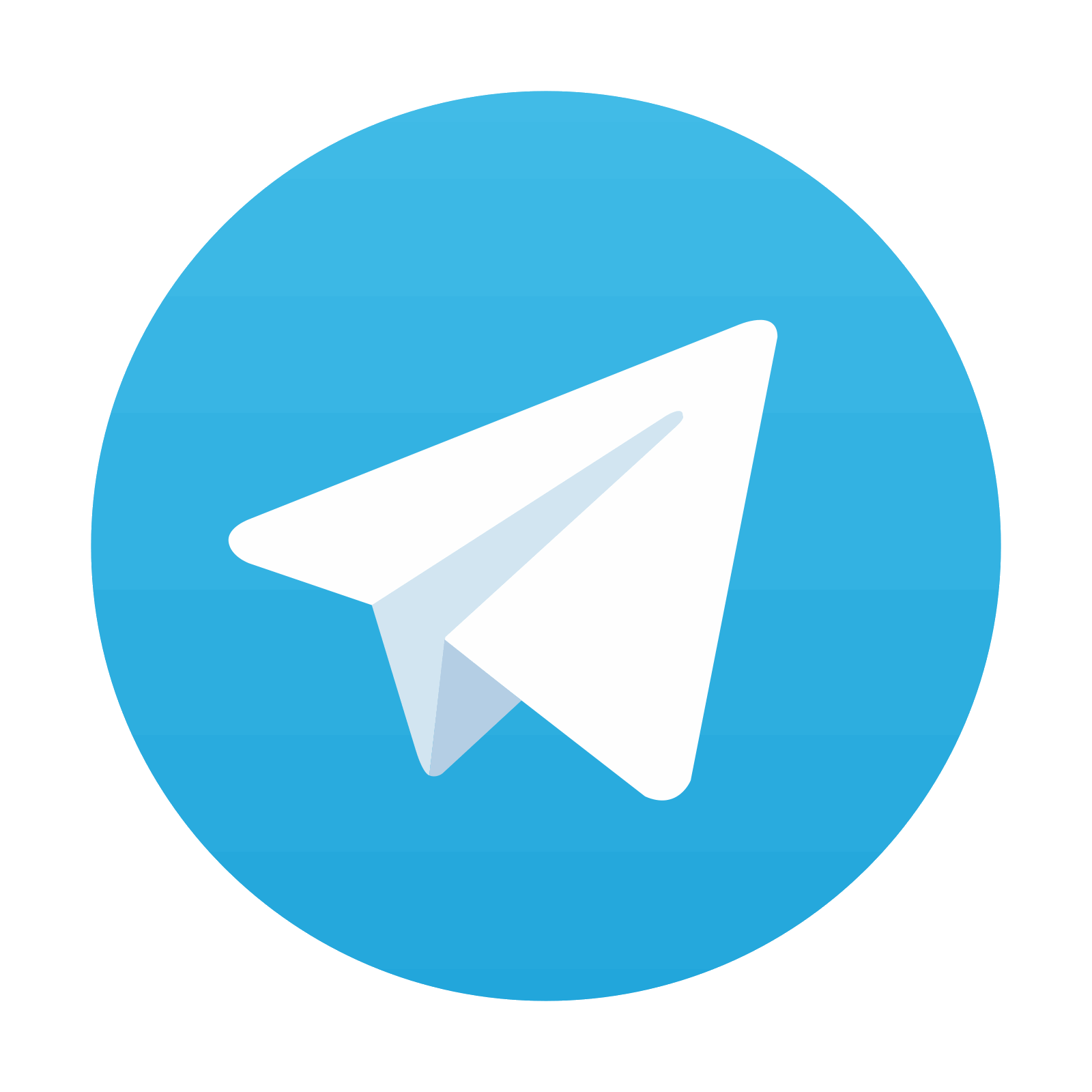
Stay updated, free articles. Join our Telegram channel
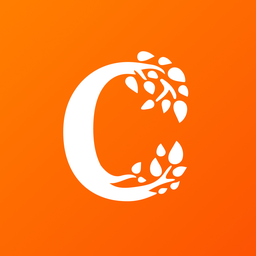
Full access? Get Clinical Tree
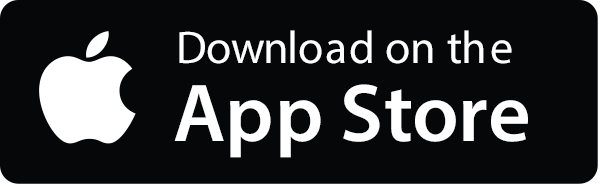
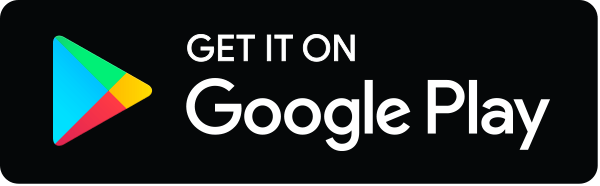