Purpose
To present the evolution of laser therapy in modern ophthalmic practice.
Design
Review of published experimental and clinical studies.
Methods
A review was undertaken of the work of multiple investigators leading to the invention of the laser, its biophysical effects on ocular tissues from which it derives its name (light-amplified stimulation of emitted radiation), and the development of various laser-based devices and methods to treat common ophthalmologic disorders, with particular emphasis on new and emerging retinal and anterior segment applications.
Results
Because the eye is optimized for the transmission of light and its transduction into neural signals, lasers are particularly well suited for ophthalmic therapy. This fact and the high demands for precision in therapy have inspired the development of highly sophisticated laser systems that have impacted the treatment of common diseases. These include diabetic retinopathy, age-related macular degeneration, retinal venous occlusive disease, retinopathy of prematurity, and optical aberrations including ametropia, cataract, and glaucoma, among others. Recent developments in scanning laser systems, including image-guided systems with eye tracking, real-time feedback, and ultra-short pulse durations, have enabled increased selectivity, precision, and safety in ocular therapy. However, improved outcomes have been associated with increased cost of medical care, and attention to and optimization of their cost effectiveness will continue to be required in the future.
Conclusions
The invention and evolution of modern ophthalmic lasers have enhanced therapeutic options and can serve as a heuristic model for better understanding the process of innovation, including the societal benefits and also unintended consequences, including increased costs.
It is a great honor to deliver the 70th Jackson Memorial Lecture and I thank the American Journal of Ophthalmology and the American Academy of Ophthalmology for selecting me to do so.
In this lecture, I discuss the invention and evolution of the ophthalmic laser over the past 5 decades. I have treated the subject not simply as a chronological listing of key milestones that have occurred over a series of decades, but rather as a narrative story about people and ideas that contributed to this process. The resulting manuscript necessarily entails a personal perspective on the field because it is drawn not only from review of the published literature and my own work in this area, but also from familiarity and professional interactions with many of the cited principals in this area over that time period.
I initially relate the early history and general principles of laser–tissue interactions as they relate to ophthalmology because they are important to understanding how lasers work and how the field evolved to the point it stands today. I then specifically focus on what I judge to be the key emerging trends that are likely to become increasingly important in the future. These include the introduction of scanning, short pulse durations, and image-guided systems. These advances have facilitated much greater precision in the dose, localization, and selectivity of laser energy delivery, as well as in automation of treatment.
Finally, there is much to be learned from studying ophthalmic laser evolution about the various aspects of medical device innovation and competition, and its impact on clinical trial design, practice patterns, and medical economics. I will attempt to touch upon these, albeit briefly. I trust that Dr Jackson, were he alive today, would have judged this a topic worthy of inclusion in this distinguished series.
The Early History of Laser
Because the eye is optimized for the transmission of light and its transduction into neural signals, it is not surprising that lasers are particularly well suited for ophthalmic therapy. The word laser itself is an acronym for a sequence of physical processes: Light Amplification by Stimulated Emission of Radiation. The prospect of stimulated emission of radiation was first predicted by Einstein in 1917, but it was another 40 years before this theoretical construct was converted into a practical device. Townes at Columbia in the late 1940s with a number of collaborators, including his postdoctoral fellow and future brother-in-law Arthur Schawlow, developed a microwave amplification chamber. During that time period, 2 Russian physicists, Alexander Prokhorov and Nicolai Basov, working apparently independently, described how a molecular beam of alkali halide directed through a resonating cavity might also result in a microwave oscillator. Townes and Schawlow, having since relocated to the Bell Labs, continued their research in amplification of increasingly shorter wavelengths in the infrared and visible spectrum, ultimately culminating in their seminal publication of 1958 on infrared and optical masers. For their efforts, Townes was jointly awarded the 1964 Nobel Prize in Physics with Prokhorov and Basov, and Schawlow separately in 1981. In 1960 Maiman at Hughes Aircraft in California produced a functioning laser for the first time with “ruby” red light output of approximately 694 nm wavelength.
The field of ophthalmic lasers evolved very quickly from that point. The technique of retinal light photocoagulation had been first pioneered 10 years earlier starting with solar coagulation by Meyer-Schwickerath in the late 1940s, and then with his work on the Xenon Arc photocoagulator in the late 1950s. The precision and selectivity of Xenon Arc coagulation, however, was limited by the fact that the light was polychromatic, the footprint of the device was large, spots were required to be very large, and the ergonomics and focusing system were difficult. As a result, although it was widely recognized that retinal light photocoagulation was promising, it remained an unwieldy and difficult technique to incorporate into clinical practice. After their invention it was soon apparent that lasers represented a better approach to light creation than xenon flash lamps, and they were rapidly embraced.
In 1961, Zaret and associates applied the pulsed ruby laser to produce lesions in the rabbit retina. Javan, Bennet, and Herriot described the first gas lasers that same year. By 1963, Kapany, Peppers, Zweng, and Flocks had expanded the field of laser retinal coagulation and demonstrated a nonlinear relationship between incident energy and burn size, and the tendency for the creation of thermomechanical effects including bubbles and retinal hemorrhage with higher energies. Additional studies with both solid-state and gas lasers soon followed by Campbell, L’Esperance and colleagues, Beetham and Aiello, and Little, Zweng and L’Esperence laying out the general principles of laser retinal photocoagulation. This included the development of a more ergonomic and precise slit-lamp delivery apparatus with articulating arm, and some of the early indications for treatment including treatment of retinal tears, diabetic retinopathy, and ocular tumors.
Ironically, although the first laser constructed by Maiman was a small solid-state prototype, the first lasers that enjoyed widespread ophthalmic adoption and commercial success were argon gas tube lasers because it was felt by many at that time that the blue-green emission spectrum of argon was better suited to the direct treatment of vascular tissues in the eye, compared with the synthetic ruby red laser light output. (H. Christian Zweng, personal communication, 1977). Gas lasers were bulkier and more difficult to operate and maintain because of the need for water cooling and fragility of gas tubes, and it was more than a decade later that more portable and economical semiconductor lasers currently in use were introduced.
Principles of Laser Light Emission
Light is composed of individual packets of energy called photons. For stimulated emission to occur more frequently than absorption and hence result in light amplification, the optical material should have more photons in an excited state than in a lower state. Such “population inversion” can be achieved using an excitation source, or “pump,” such as a flash lamp or electrical source that imparts the energy to achieve population inversion into the cavity. In that cavity, emitted light circulates between 2 mirrors on either side, with a fraction of the photons escaping through an aperture in 1 of the 2 mirrors, which is semi-reflective, to form the laser beam. The light trapped inside the cavity stimulates emission of new photons from the excited laser material with the same wavelength, direction, and phase, thereby forming a coherent laser beam, as illustrated in Figure 1 . This beam can then be directly emitted into space, focused with an appropriate lens system, transmitted by a fiber-optic cable or some combination of the above to achieve the desired effects.
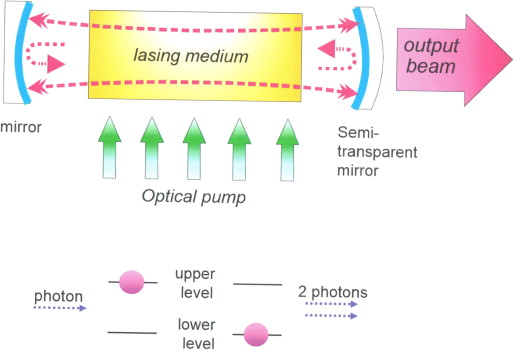
Principles of Laser Light Emission
Light is composed of individual packets of energy called photons. For stimulated emission to occur more frequently than absorption and hence result in light amplification, the optical material should have more photons in an excited state than in a lower state. Such “population inversion” can be achieved using an excitation source, or “pump,” such as a flash lamp or electrical source that imparts the energy to achieve population inversion into the cavity. In that cavity, emitted light circulates between 2 mirrors on either side, with a fraction of the photons escaping through an aperture in 1 of the 2 mirrors, which is semi-reflective, to form the laser beam. The light trapped inside the cavity stimulates emission of new photons from the excited laser material with the same wavelength, direction, and phase, thereby forming a coherent laser beam, as illustrated in Figure 1 . This beam can then be directly emitted into space, focused with an appropriate lens system, transmitted by a fiber-optic cable or some combination of the above to achieve the desired effects.
Laser–Tissue Interactions
Interactions of light with biological tissues depend on its wavelength, pulse duration, and irradiance (amount of power per unit area, W/cm 2 ). With sufficiently high photon energy, light can induce photochemical reactions. Light absorption by the tissue can also result in heating, which may lead to thermal denaturation. At higher temperatures, and especially with shorter pulses, tissue can be rapidly vaporized, which can result in mechanical disruption or tissue ejection. Very short pulses with high peak irradiance can ionize materials, enabling precise dissection of tissue. These types of interactions are described below in more detail.
Photochemical Interactions
Light-induced chemical reactions are typically not associated with a meaningful change in tissue temperature. Such interactions are typically mediated by exogenously administered agents, such as verteporfin. Photochemical interactions are the basis for retinal photodynamic therapy in age-related macular degeneration, or cross-linking of corneal collagen with riboflavin to treat keratoconus. To avoid heating, therapeutic photochemical interactions are typically performed at very low irradiances (<1 W/cm 2 ) and with long exposures—tens of seconds or minutes.
Photothermal Interactions
Depending on the temperature rise and duration of exposure, different tissue effects may occur, including metabolic alterations, necrosis, and vaporization. The absorption coefficient of biologic tissue chromophores strongly depends on the laser wavelength. Major chromophores of ocular tissues include water, proteins, melanin, blood, and macular pigments. The extent of tissue damage can be quantified by the Arrhenius integral Ω, which describes the changes in temperature in time and space in biologic tissue in response to the application of laser energy. This relationship reflects the rate at which different individual proteins with important structural and regulatory functions in a cell are affected in response to laser treatment. Denaturation of cellular proteins varies as an exponential function of temperature and a linear function of the laser pulse duration; the Arrhenius integral mathematically describes this relationship. The parameters necessary to create lesions of differing effect in biologic tissue of defined composition can be chosen based on the predicted decline in the concentration of different critical protein molecular components of the cell necessary for normal structure and function. This may range from visually imperceptible events such as induced cytokine expression without obvious structural change to frank apoptosis, necrosis, or vaporization of cells, depending on the amount and temporal profile of energy applied. Clinical examples of this are illustrated in the subsequent section on new approaches.
Retinal Photocoagulation
Retinal photocoagulation typically involves application of pulses with durations ranging from 10 to 200 ms, and transient hyperthermia by tens of degrees Celsius above body temperature. Various lasers have been used in the past, including ruby (694 nm), argon (488, 514 nm), and krypton (647 nm). Currently the most common lasers in photocoagulation are green frequency-doubled neodymium–yttrium-aluminum-garnet (Nd:YAG) (532 nm) and yellow semiconductor lasers (577 nm). The laser energy is absorbed primarily by melanin in the retinal pigment epithelium (RPE) and choroid, and by hemoglobin. Heat generated by light absorption in the RPE and choroid diffuses into the retina and causes coagulation of the photoreceptors and, sometimes, of the inner retina. During 100 ms applications, the heat diffuses up to 200 μm, thus “smoothing” the edge and extending the coagulated zone beyond the boundaries of the laser spot, termed “thermal blooming.” Heat diffusion using shorter pulses and smaller spot sizes can be limited to the RPE and photoreceptor layers, thereby avoiding or minimizing inner retinal damage. The diameter of the acute retinal lesion increases logarithmically with increasing pulse duration ( Figure 2 , Top) and linearly with increasing laser power ( Figure 2 , Bottom).
The threshold power required for the creation of a retinal lesion increases with decreasing pulse duration. A relatively modest power increase is required to produce comparable lesion grades going from 100 ms to 10 ms, whereas a much steeper increase is seen for durations under 10 ms. At 2 ms or less it is not consistently possible to reproducibly create a visible lesion of moderate grade without rupturing the retina, and therefore shorter pulse durations in this range or lower should never be used to create visible lesions, although they can be used safely when the desired treatment endpoints are sub-visible.
When only the outer layers of the retina are acutely damaged with shorter pulse durations ( Figure 3 , Bottom left) compared with the full-thickness burns seen acutely with longer durations ( Figure 3 , Top left), the retina shows considerable capacity for renewal through regeneration and remodeling. RPE migration and proliferation restore continuity of the RPE monolayer within 1 week compared with full-thickness damage with longer-duration pulses of 100 ms. The damage zone in the photoreceptor layer is initially filled with glia. Over time, the photoreceptors from the adjacent retina shift into the damage zone, thereby reducing its size. With sufficiently small lesions (below 200 μm), and no damage to the inner retinal layers, photoreceptors can completely refill the damage zone and rewire to local bipolar cells over time, thereby restoring retinal structure and function (Bottom right) and avoiding the extensive glial scarring and neuronal loss associated with longer-duration retinal burns ( Figure 3 , Top right). These effects can be modulated with the concomitant administration of pharmacologic agents such as steroids.
Photomechanical Interactions
Photoablation and Photodisruption
Photomechanical interactions are at the heart of laser in situ keratomileusis (LASIK) and other types of corneal refractive surgery. Precise corneal photoablation is achieved using nanosecond pulses of ArF excimer laser as a result of the limited 200 nm penetration depth of 193 nm radiation in the cornea and very short pulse durations (∼10 ns) enabling extremely precise ablation, with a very narrow zone of residual tissue damage (<0.2 μm) at the edges of the ablation zone. Both photoablation and photodisruption occur when laser absorption results in the tissue temperature exceeding the vaporization threshold. Expanding and collapsing vapor bubbles following explosive vaporization can rupture nearby tissue or eject tissue fragments from the exposed surface. Vaporization temperature ranges between 100 and 305 C. To avoid heat diffusion away from the laser absorption zone during the pulse, energy needs to be applied using relatively short pulse durations—in the range of microseconds to nanoseconds rather than milliseconds.
Dielectric Breakdown
At extremely high irradiances (10 8 –10 11 W/cm 2 ), which can be achieved in a tightly focused short-pulsed (ns-fs) laser beam, the electric field is so high that even transparent material can be ionized. This mechanism, called dielectric breakdown, allows for a highly localized deposition of energy at the focal point of the laser beam. The development of plasma and associated absorption of the laser energy in the focal spot lead to explosive vaporization of the liquid medium, accompanied by tissue rupture.
Plasma-mediated laser–tissue interactions are applied to fragmentation of an opacified posterior lens capsule with nanosecond Nd:YAG lasers. At shorter pulse durations (1 ps to 100 fs) and much lower energies, this process is applied to intrastromal cutting for refractive surgery. This approach has also been tested in the dissection of epiretinal membranes using a tightly focused beam. Despite the very low energy required for this process, its applicability in the posterior pole is limited owing to the difficulty in axial differentiation between the epiretinal membranes and the retina located very close behind them. Recently, this process has been applied to anterior capsulotomy and fragmentation of the crystalline lens during cataract surgery.
Major Clinical Indications for Ophthalmic Laser Therapy
Vitreoretinal Diseases
Laser has been a mainstay for the treatment of a large number of vitreoretinal diseases in the years since its introduction. The major conditions include diabetic retinopathy, age-related macular degeneration, retinal venous occlusive disease, retinal breaks and detachment, ophthalmic tumors, and miscellaneous other retinal and choroidal vascular conditions including central serous choroidopathy, sickle cell retinopathy, and retinopathy of prematurity; these are well covered in many prior publications. The Diabetic Retinopathy Study (DRS) and Early Treatment of Diabetic Retinopathy Study (ETDRS) were pivotal events in the history of clinical research, as 2 of the first large extramural multicenter trials supported by the National Institutes of Health to assess the safety and efficacy of new technologies. At the time, laser was still a somewhat controversial therapy prior to the release of the study results. A long-term benefit of these studies was their contribution to establishing the large federally sponsored prospective multicenter randomized trial as the gold standard for evaluation of new forms of ophthalmic therapy. Focal and grid laser today, either alone or in combination with pharmacologic agents including ranabizumab, bevacizumab, and intravitreal steroids, still play an important role in the control of macular edema for a large number of patients, including those with more focal disease and leakage or those not responsive to pharmacologic agents alone as well as selected cases of macular edema associated with retinal vein occlusion.
One of the great remaining mysteries of retinal photocoagulation is the precise mechanism by which panretinal photocoagulation (PRP) works to cause regression of retinal and iris neovascularization, and the effect of grid photocoagulation on diffuse capillary leakage in diabetic retinopathy and retinal venous occlusive disease. Most theories that have been advanced center around reducing expression of vascular endothelial growth factor (VEGF). However, the way in which laser accomplishes this remains unknown, and may involve upstream effects including facilitation of transport of nutrients, including oxygen, to the retina from the choroid; transport of by-products of metabolism out of the retina; overall reduction of retinal metabolic load by decreasing the total number of photoreceptors; or reduced sequestration of proangiogenic cytokines in the photoreceptors. There may be a secondary, although not fully understood, benefit from less damaging treatment of the retina whereby this treatment promotes secretion of cytokines that downregulate angiogenesis and capillary permeability. Possible cytokines involved in this pathway include heat shock protein and alpha-crystallins, as well as other complexes, and they may impact gene expression that ultimately affects inflammation and hyperpermeability in the retina.
Cataract and Refractive Disorders
Q-switched Nd:YAG lasers were first introduced by Aron-Rosa and associates and Fankhauser to noninvasively perform posterior capsulotomy following cataract surgery. New photomechanical lasers for corneal applications were subsequently introduced, beginning with the introduction of the argon fluoride excimer laser, which was capable of producing smooth surface ablation of tissue with extremely higher radiances and ultrashort focused pulses in the range of nanoseconds to femtoseconds. Further refinements in excimer laser–assisted corneal refractive surgery included the technique of laser-assisted LASIK, a technique that has largely replaced photorefractive keratectomy owing to fewer effects on the corneal epithelium. This procedure is typically now performed with the use of an intrastromal femtosecond laser, rather than the mechanical microkeratome originally described, for cutting of the corneal flap. Photodisruptive lasers have also been explored as a means to improve the elasticity of the aging lens to counteract presbyopia, although none have yet been proven effective or commercialized.
Recent Innovations in Ocular Laser Therapy
The Impact of Scanners
The availability of scanning galvanometers has had an important impact on the creation of sophisticated medical laser delivery systems, capable of increased efficacy, precision, and safety. Initially both retinal and anterior segment lasers employed single pulses, or trains of pulses, which limited the area of tissue that could be treated and also the ability to easily differentiate treated from untreated regions within that area, depending on the clinical need and availability of visible endpoints.
In the case of excimer refractive lasers, the first Food & Drug Administration (FDA)-approved laser employed the method of broad beam ablation, which limited the physician’s ability to easily treat patients with astigmatism, or other higher-order optical aberrations, with customized ablations. This limitation was resolved with the introduction of scanning excimer lasers that were capable of treating relatively larger areas of the cornea by rapidly moving the beam using a computerized system that determined the area and depth of ablation and could be individualized based on preoperative measurements.
In the case of retinal lasers, the decision to use scanners was initially driven by our interest in reducing treatment times for patients undergoing diabetic retinopathy management but ultimately resulted in a number of other unanticipated benefits. In order to use a scanner, it was necessary to shorten each individual pulse duration from the DRS and ETDRS standard of 100–200 ms. We chose 10–30 ms pulse durations as a new standard in order to ensure that an array of multiple burns could be placed precisely where intended and not be influenced by ocular movements that typically occur with time durations in excess of 200 ms. Although the original intent was to insure that these shorter-duration pulses resulted in lesions histologically comparable to longer-duration burns, we soon recognized that the use of shorter pulse durations resulted in a greater ability to predict the precise depth of impacted, including impacted tissue minimizing the amount of collateral damage.
The shorter-pulse-duration burns appeared more uniform and smaller than lesions induced by traditional longer pulse durations. While it had been previously shown that laser pulse durations in the range of nanoseconds to microseconds were capable of limiting the damage to the pigment epithelium, for sub-visible burns, this same precise effect on selective layers of the retina had not been well characterized for visible burns. Additionally, the clinical utility and acceptance of this type of sub-visible therapy by clinicians had been limited by the inability to reliably judge where to place spots relative to other earlier sub-visible burns during treatment sessions. With the introduction of scanners, many of the limitations of single spot lasers, whether small or broad beam, were resolved and new opportunities presented. These included a more complete examination of the role of laser systems with short (tens of milliseconds) as well as ultra-short (microseconds) pulse duration and new methods of photocoagulation.
Short-Pulse-Duration and Reduced-Fluence Systems
Choices in the treatment parameters of pulse duration, power, wavelength, and aerial spot size have a predictable impact on the intensity, depth, and ultimate size of coagulative lesions, as correctly predicted by the Arrhenius model described above. These impacts have served as the basis for the development of a new generation of commercial retinal lasers, including those that function in both the millisecond and microsecond domain with and without scanning and image-guidance capability. Pattern scanning lasers with typical pulse durations in the range of 10–30 ms produce burns primarily confined to the pigment epithelium, photoreceptors, and outer retina. As a result, secondary complications, including visual field defects associated with ganglion cell loss and nerve fiber layer defects, as well as pain from choroidal heating, occur less frequently.
Further reduction in the pulse durations to below 50 μs enables selective photodisruption by explosive vaporization of the melanosomes in the RPE, sometimes termed selective retinal therapy (SRT). Since heat does not escape from the melanosomes during microsecond pulses, there is sparing of photoreceptors and Bruch membrane. In a few days RPE cells from adjacent areas migrate into the damage zone and fill the defects, thereby restoring the RPE monolayer, so it can again support the metabolic needs of the photoreceptors that remain viable above the treated zone. While the lesions may not be visible at the time of application, they may be either subsequently delineated by the passage of time and atrophic scar formation or demonstrated acutely by enhanced imaging techniques, including both retinal fluoroscein angiography and optical coherence tomography (OCT) examination.
An alternative approach to the use of individual pulses is the application of patterns of relatively short pulse durations, in the range of single milliseconds, which can be applied stepwise as a semiautomated grid. With this technique, the patterns of sub-visible burns can be outlined by slightly more intense marker burns delineating the boundaries of the treated area. This pattern scanning approach to treatment can use laser settings that create structural damage to selected layers, such as photoreceptors or just the RPE, or less energetic nondamaging hyperthermia in which the only effects are thought to be metabolic, as evidenced by the lack of structural changes in histologic and contrast imaging studies.
The laser energy can be optimized in accordance with the Arrhenius integral model, to select a threshold power and pulse duration necessary to create a sub-visible non–structurally damaging tissue effect owing to the lower tissue temperatures produced. As has been shown in prior laboratory studies, the peak tissue temperature needed to stimulate expression of heat shock protein 70, a naturally occurring ubiquitous cytokine that inhibits inappropriate protein aggregation, inflammation, and apoptosis, is 49 C. At that temperature point, no structural damage is seen in photocoagulated tissues in experimental models, compared with the 62 C temperature that typically results in cell death. With this approach 200 μm spots may be placed at 30% of the energy needed to produce a barely visible burn using green or yellow laser light and a grid pattern with 0.25 beam diameters spot spacing covering the area of abnormal retina where there is accumulation of subretinal or intraretinal fluid. Although initial clinical experience with this approach is still limited, it appears that a number of patients treated with this regimen may show clinically significant benefits, including resolution of intraretinal and subretinal fluid and improvement in visual acuity in cases of diabetic macular edema, central serous chorioretinopathy, and branch retinal vein occlusion ( Figure 4 ). As of yet there have been no published large randomized multicenter trials employing this approach, although the initial experience has been sufficiently promising that planning efforts are underway to undertake such trials. Such randomized studies are required to confirm the utility of this approach to larger groups of patients.
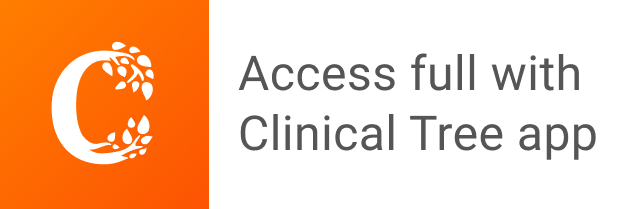