The Anatomy and Cell Biology of the Retina
Susanna S. Park
Interest in retinal anatomy has increased in recent years with the understanding of the importance of retinal function in the visual system. Historically, the retina was first described by Herophilus of Chalcedon (circa 300 B.C.E.) and named as such by Rufos of Ephesus (circa 110 C.E.) because of its appearance as a net to hold the vitreous humor. Despite Galen’s early observation of the similarity between this tissue and the brain, little attention was directed to the retina beyond its obvious vasculature and connection to the optic nerve until Kepler (circa 1608) introduced the concept of the retina as the primary photoreceptor tissue of the eye.
With the technical advancement in alcohol tissue fixation, Treviranus first performed the detailed microscopic studies of retinal anatomy in 1835. The later development of thin tissue sections and staining techniques allowed the study of complete neurons and the development of a tentative understanding of the neuronal cell polarity and wiring. The recent use of electron microscopy, immunohistochemistry, and single-cell electrophysiologic studies furthered our understanding of the intraretinal cellular connections and ultrastructure and their role in the complex electrical processing of visual information. New clinical imaging techniques, such as optical coherence tomography, allow us to examine retinal histology in vivo, thus advancing our understanding of pathogenesis of various retinal disorders.
The first detailed study of the anatomy of the retinal vasculature was possible with the advent of the trypsin digest technique. This provided an initial framework for the understanding of retinal vascular physiology. More detailed understanding of the retinal vasculature has been possible recently with progress in whole-mount tissue preparations. Clinical use of fluorescein angiography and newer techniques of vascular flow measurements, e.g., scanning laser Doppler flowmetry, have made possible the correlation between in vivo physiologic vascular changes and previously observed histologic changes in various pathologic entities.
This intimate relationship between the development of concepts of retinal structure and function is no accident. Discoveries in each of these areas have been the basis for further investigation into the other. This chapter is an overview of our current understanding of the retinal anatomy, at both gross and microscopic levels. When appropriate, anatomic correlation is made with various physiologic and pathologic retinal processes.
GENERAL ANATOMY
The retina is a diaphanous, transparent tissue that lines the inner posterior three-fourths of the eyewall.1 It extends from the macula in the posterior pole to a location approximately 5 mm anterior to the equator, the ora serrata, where it becomes contiguous with the nonpigmented epithelium of the pars plana ciliaris. It is loosely adherent to the underlying pigment epithelium, and the two tissue layers can easily be separated in postmortem specimen. The only firm attachments of the retina are at the margins of the optic disc and at the ora serrata (Fig. 1). In living eyes, the retina also is attached to the overlying vitreous in a circular band around the retinal periphery, referred to as the vitreous base. Other points of attachment between the retina and the vitreous may exist around the optic disc and in the macular region.
Gross retinal topography reveals characteristic regional variations. The clinical posterior pole is the central posterior retina approximately 5 to 6 mm in diameter, lying between the two temporal retinal arteries (Fig. 2). It is referred to anatomically as the area centralis or macula. Histologically, it is the only region of the retina with more than one layer of ganglion cells. The clinical macula, or the anatomic fovea, is the central area approximately 1.5 mm in diameter within the area centralis. The name macula lutea was given to this region because of the slightly yellow coloration of this area resulting from the presence of xanthophyll in the ganglion and bipolar cells.2 Within the center of the anatomic fovea lies a depression approximately 0.35 mm in diameter surrounded by a ring of slightly thickened tissue. This region is called the foveola by anatomists and the fovea by clinicians. It lies within the capillary-free zone, which measures approximately 0.4 mm in diameter in most people. The photoreceptors in this region characteristically consist of pure cones. The center of the pit within the foveola is called the umbo. It is located 3.4 ± 0.34 mm from the temporal edge of the disc margin and 0.8 mm inferior to the center of the optic disc.3 The anatomic fovea is surrounded by a 0.5-mm-wide ring zone called the parafovea. This area is where the ganglion cell, intranuclear layer, and outer plexiform layer are the thickest. A 1.5-mm ring zone surrounding the parafovea is called the perifoveal area.
![]() Fig. 2 Posterior retina with comparative anatomic and clinical terminologies. (Redrawn from Patz A: Principles of fluorescein angiography. Int Ophthalmol Clin 17:4, 1977) |
Beyond the area centralis, the retina spreads out smoothly over the vortex veins, which are visible through the retina and underlying pigment epithelium during ophthalmoscopy. This equatorial region lays approximately 15 mm posterior to the corneoscleral junction and approximately 3 mm posterior to the geometric equator in the emmetropic globe.
At the equator, the retina has a vertical diameter of 24.08 ± 0.94 mm and a horizontal diameter of 24.06 ± 0.60 mm. Measured as a cord, the distance from the corresponding margin of the optic disc to the equator is 14.71 ± 1.08 mm in the superior meridian, 14.51 ± 1.01 mm inferiorly, 13.27 ± 1.11 mm nasally, and 17.29 ± 1.60 mm temporally. This diameter provides a theoretic estimate of the retinal area as 1206 mm2 (estimating the retinal surface to cover two thirds of a sphere of this dimension). Changes in tissue size and shape during preparation have precluded the estimation of the actual retinal area.
Anteriorly, the retina can be seen to terminate in an irregularly wavy edge, the ora serrata. Measured as a cord from the equator, this lies 6 ± 1.22 mm temporally, 5.8 ± 1.12 mm nasally, 5.07 ± 1.11 mm superiorly, and 4.79 ± 1.22 mm inferiorly. Using 1.50 mm as the estimation of a disc diameter (dd), the distance from the equator to the ora is approximately 3 dd. The distance from the anterior limit of the retina to Schwalbe’s line is 6.14 ± 0.85 mm superiorly, 6.20 ± 0.76 mm inferiorly, 5.73 ± 0.81 mm nasally, and 6.52 ± 0.75 mm temporally.4 This places the ora approximately at the point of the rectus muscle insertions around the globe. A recent study of the relationship between the ora serrata and the spiral of Tillaux in cadaver eyes showed that in 68% the midpoint insertion of all rectus muscles was within 1 mm of the ora serrata.5 In addition, in most cases, the lateral rectus muscle most consistently inserted closest to the ora.
This anatomic fact is important in vitrectomy surgery. The surgeon can place the sclerostomies for pars plana vitrectomy as far back as 4 mm posterior to the limbus and still have access to the ora serrata extending circumferentially from the meridian of the sclerostomy.6 The 95% tolerance interval for the location of the ora serrata is 4.21 mm posterior to the surgical limbus superiorly and at least 4.81 mm posterior to the limbus temporally.5
The termination at the ora is marked by retinal extensions, labeled teeth, which protrude into the ciliary epithelium. The teeth are more prominent in hyperopic than in myopic eyes, and they have an uneven but characteristic distribution around the ora. Separating the teeth are ora bays, which are indentations of the ciliary epithelium onto the retina. The vitreous base is attached to the retina at the ora from approximately 1.8 mm temporally to 3 mm nasally, posterior to the ora bays.7 The anterior insertion of the vitreous base into the ciliary epithelium is 1 or 2 mm anterior to the ora. The posterior margin of the vitreous base has a relatively smooth contour in normal young eyes, whereas the anterior margin follows the serrations. With aging, the posterior margin of the insertion becomes irregular and migrates posteriorly such that it is close to the equator.8 These changes are thought to play a role in the pathogenesis of retinal breaks from peripheral retinal traction.9
A cross section of a low-power histologic preparation of the retina in the region immediately peripheral to the area centralis shows it to have nine layers (Fig. 3). From internally (the side toward the vitreous), the layers are as follows:
The internal limiting membrane
The nerve fiber layer
The ganglion cell layer
The inner plexiform layer
The inner nuclear layer
The outer plexiform layer
The outer nuclear layer
The rod and cone inner and outer segments
Retinal pigment epithelium
Based on histologic studies, the retina is thickest at the foveal margin (0.23 mm) and tapers to approximately 0.10 mm at the foveal center (umbo) and 0.18 mm at the equator. It gradually thins further to 0.11 mm in thickness at the ora serrata (Fig. 4A) as the density of all the neuronal elements, including photoreceptors and ganglion cells, decreases peripherally.3 With use of noninvasive techniques in normal live human subjects, the mean thickness of the foveal center is estimated to be 0.18 mm using the retinal thickness analyzer and 0.15mm with optical coherence tomography (Fig. 4B).10 Among patients with clinically diagnosed macula edema, the mean foveal thickness increases to 0.40 mm using the retinal thickness analyzer and 0.32 mm with optical coherence tomography.
The distinct layering pattern of the retina reflects the arrangement of neural, glial, and vascular cells that enables the retina to convert and process photic energy into neuronal electrical signals that ultimately reach the occipital visual cortex of the brain. This entire pathway consists of the photoreceptor cell and three neurons.11 The photoreceptor cells (rods and cones) are located in the outermost layer of the retina. The outer segments of the rods and cones initially convert the light signal to electrical energy. The outer nuclear layer contains the cell bodies with nuclei of the photoreceptor cells. In the outer plexiform layer, axons of the photoreceptor cells synapse with the dendrites of the first neuron (the bipolar cell) and with the processes of the integrating neuronal cells, the horizontal cells. The bipolar cells, whose nuclei form the bulk of the inner nuclear layer, synapse in the inner plexiform layer with the dendrites of the second neurons, the ganglion cells. In this layer there are also synapses with amacrine cells, which resemble horizontal cells in that they provide cross wiring within the retinal layer. The ganglion cells extend their axons as the innermost retinal layer, the nerve fiber layer, through the optic nerve to the lateral geniculate body, where they synapse with the third neuron, the cells of the lateral geniculate body, which extend their axons through the optic radiations to the occipital cortex (Fig. 5).
TOPOGRAPHY OF THE RETINA
FOVEA
In the region of the anatomic fovea, the retinal layers have a unique arrangement specialized for color vision and better visual acuity than in the remainder of the retina. The entire fovea measures 1.5 mm in diameter and contains 10% of the cones. It has the highest cone density in the retina, approximately 147,300 per square millimeter.12 The central 0.40-mm zone is free of capillaries and is nourished by the choriocapillaris circulation.13 Clinically, this region is referred to as the foveal avascular zone and can be readily visualized on fluorescein angiography (Fig. 6).
In the center of the fovea is a specialized structure 0.33 mm in width, the foveal pit or foveola (Fig. 7).13 This central-most region is free of rods and blue cones (Fig. 8). The red and green cones in this region are oriented parallel to each other and are perfectly straight and vertical with respect to the retinal surface to allow the greatest light sensitivity. The inner segments of these cones are separated from each other by Müller cells.14 In addition, the foveal cones differ from cones elsewhere in the retina. They are long and slender like rods, measuring approximately 80 μm in height. In addition, these cones have a large nucleus and lack the dense collection of mitochondria seen in the ellipsoid of most photoreceptors.
To minimize the light scatter through the tissue overlying the cones, the inner central fovea is composed only of processes of the Müller cells. It lacks the inner nuclear layer, inner plexiform layer, ganglion cells, and the nerve fiber layer. In addition, the external plexiform layer has a unique configuration in the anatomic fovea. The external cone fibers (axons) at the foveola make an almost right angle turn away from the foveola and assume a course parallel to the surface of the retina. From the parafoveolar region to the edge of the anatomic fovea, the fibers of the remaining cones and of the rods pursue a similar course. The aggregate of all these horizontally arranged fibers forms Henle’s layer of the anatomic fovea or clinical macula (Fig. 7B). After a short course parallel to the retinal surface, these fibers become perpendicular to the retinal surface to synapse with the dendrites of the overlying bipolar cells. This first synapse between the photoreceptors and bipolar cells of the fovea occurs outside the central 0.2-mm diameter of the foveola.14
Clinically, this region has a yellow discoloration on funduscopy because of the presence of xanthophyll in the ganglion and bipolar cells. This macular pigment has several proposed functions, including prevention of chromatic aberration at the fovea, selective absorption of potentially toxic blue light, and quenching of various active oxygen species.15 In the human retina, two components of the macular pigment have been identified.16 They are zeaxanthin and lutein, two structural isomers of dihydroxyxanthophyll. In general, zeaxanthin is confined mostly to the foveal region, whereas lutein is found more widely distributed in the posterior pole. Studies on the monkey retina suggest that a particular ratio of these two xanthophylls may be associated with a specific cone subtype.17 Among patients with age-related macular degeneration, decreased levels of these pigments have been noted in the macula.18 Because these pigments are potent antioxidants, they may play an important role in maintaining the health of the retinal cells in the macula during aging. In animal models, they have been shown to protect photoreceptors from light-induced apoptosis.19
PARAFOVEA
The parafovea, defined as the 0.5-mm-wide annular zone surrounding the fovea (for a total diameter of 2.5 mm), has the largest accumulation of neurons in the entire retina. The bulk of these cells are in the ganglion and inner nuclear layers. This is also the region where the outer plexiform layer forms the thickened Henle’s layer by the multitudinous axons of the foveola photoreceptors (see Fig. 7A). This region has a lower density of cones than at the foveolar center. There are approximately 100 cones per 100 μm, and the cone inner segments are separated from each other by 1 to 3 μm.14 The rods also begin to appear in this region, and toward the peripheral edge of this parafoveal zone the outer nuclear layer becomes filled mostly with rod nuclei. Immediately temporal to the papilla, the parafovea contains the highest rod density, 170,000 per square millimeter.12 The inner nuclear layer here is also very dense and consists of up to 12 rows of cells. Similarly, the ganglion cell layer forms up to seven rows of closely packed cells at the foveal edge. The nerve fiber layer is thickest in the region of the papillomacular bundle nasal to the fovea and is thinner temporal to the fovea.
PERIFOVEAL REGION
The perifoveal region is the outermost ring of the anatomic area centralis. It starts at the point where the ganglion cell layer has four rows of nuclei and ends peripherally where the ganglion cell layer is reduced to the single-cell layer, typical of the peripheral retina. Funduscopically, the perifovea includes the circular area 1.25 to 2.75 mm from the foveolar pit. It defines the periphery of the clinical macula, which has a horizontal diameter of 5.5 mm and corresponds to a visual field of 18.3 degrees.
The perifovea differs from the parafovea in that it has a less densely packed array of cone outer segments. The rod density is increased to an average of two rods between adjoining cones. The outer nuclear layer of the perifoveal zone, similar to that of the peripheral parafovea, contains the maximum density of rods. However, the outer plexiform layer and the inner nuclear layer are reduced in thickness in this zone compared with those of the parafoveal zone. This is because the perifoveal outer plexiform layer does not carry the axons of the cones in the foveola. The inner plexiform layer also is reduced to six or seven rows of nuclei as the bipolar cell density decreases away from the parafoveolar zone. In contrast, the inner plexiform layer is slightly thickened, and the ganglion cells are somewhat larger in the perifoveal zone than in the parafovea.
ORA SERRATA
The peripheral edge of the retina is the ora serrata. It marks the junction between the multilayered pars optica retinae and the monolayered nonpigmented epithelium of the ciliary body. The distinct anatomic properties of this region are because of its thinness, lack of vascularity, and intimate relationship to the vitreous base and zonular fibers. The ora serrata receives its name because the retina has marginal notches over much, if not all, of its border with the ciliary epithelium. They are composed of elongations of the retinal tissue into the nonpigmented ciliary epithelium and are known as dentate processes or, more simply, teeth. The excavations between the teeth, which have their convexity posteriorly, are designated as bays. The width of the zone from the tips of the ora teeth to the back of the bays ranges from 2.1 mm on the temporal side of the retina to between 0.7 and 0.8 mm nasally.20
The distribution of ora teeth and bays around the retinal circumference is uneven. The highest density of teeth occurs in the inferonasal quadrant, and the lowest density occurs in the inferotemporal quadrant.3 The number of dentate processes ranges from 16 to 48 among eyes but averages between 25 and 30.20 The processes are longer and thereby more prominent in emmetropic and hyperopic globes than in myopic globes. The teeth are oriented radially with the points aimed between valleys of the ciliary processes. The bays between the teeth may assume various configurations, depending on the depth of the bay and the prominence of the neighboring teeth. The bay may range from only an indentation in the ora to a fully enclosed profile that is surrounded on all sides by retinal tissue. These enclosed bays are anomalies of development and do not change with aging.
The ora teeth may show developmental variations in size and shape.3,21 A regular dentate process is a retinal extension that projects 0.5 to 2.5 mm anterior to the adjacent retina on both sides. In contrast, a large dentate process protrudes onto the pars plana of the ciliary body more than 2.5 mm anterior to the surrounding retina. A giant dentate process extends to or onto the pars plicata of the ciliary body. When a tooth shows thickening by virtue of focal hyperplasia and hypertrophy of its glial component, a solid retinal tuft is evident (Fig. 9). Such a lesion is present in 33% of the adult population. Cystic retinal tufts reflect the same congenital structure as that of the solid tufts, but they are more rare and have a cystic space that results from cavitation within the glial mass (Fig. 10). These lesions have overlying vitreous condensations and may be avulsed by vitreous traction. They occur in approximately 5% of the adult population and may account for 10% of cases of rhegmatogenous retinal detachment. A meridional fold (Fig. 11) is the result of glial overgrowth along a radial line extending anteriorly from behind the base of the tooth to its tip. This is a normal developmental variation that occurs in 26% of the population. A characteristic common to all of these hypertrophic developmental anomalies, except cystic retinal tufts, is that they are benign.
When a dentate process occurs in the same meridian as a ciliary process, a “meridional complex” is formed (see Fig. 11). In this abnormal alignment, the dentate process is exceptionally large and usually combined with a meridional fold. It is continuous with the enlarged ciliary process and often is associated with a peripheral retinal excavation in the corresponding meridian. Both the large dentate process and meridional fold are composed of excessive, disorganized, and somewhat degenerated retinal tissue. Meridional complexes are present in 16% of the population and are bilateral in 58% of affected patients. They are most common in the superonasal quadrant and are multiple in 45% of affected eyes.22
Enclosed and partially enclosed ora bays (Fig. 12) are relatively uncommon developmental variations.23 They are oval islands of pars plana epithelium located immediately posterior to the ora and completely or almost completely enclosed by peripheral retina. These abnormal formations are seen in only 6% of people. In 8% of affected patients, they are bilateral. They are present in 3% of all eyes.22 These formations tend to have the greatest prevalence near the horizontal meridians both nasally and temporally. In 20% of the cases, a meridional fold is found immediately anterior or posterior to an enclosed ora bay.24 The clinical significance of the enclosed bays is that they are often mistaken for a retinal hole. In addition, the posterior edges of enclosed ora bays have an abnormally high rate of retinal tear. After a posterior vitreous detachment, 16% of enclosed or partially enclosed ora bays have an associated retinal tear along the posterior edge.23 This results from the relatively weaker bond of the retina to the underlying pigment epithelium at the edge of the bay as compared with the stronger bond of the retina to the nonpigmented ciliary epithelium. This difference puts excessive traction on the retinal tissue at the posterior margin of the enclosed bay.
The ora is approximately 0.11-mm thick and is the thinnest part of the retina outside the fovea (see Fig. 4A). Further thinning within the ora may occur as discrete pits (see Fig. 12). Such pits are frequently aligned with a meridional fold or meridional complex.22 The location of these pits varies from 1 to 7.2 mm posterior to the ora. Their formation is caused by the focal loss of the inner retinal layers (Fig. 13). The tissue surrounding the pit is normal. Such peripheral retina excavations are present in 15% of patients. In 43% of affected persons they are bilateral. Half of the affected eyes contain two or more areas of focal excavation, most commonly in the superior nasal quadrant. Because of an abnormal insertion of vitreous fibers into the retina in meridional folds and complexes associated with pits, the posterior edge of a meridional complex is a common site for a retinal tear. In addition, traction on the retina in the area of a pit could easily produce a full-thickness perforation because of the extreme thinning of the retina at this point.
The relationship of the peripheral retina to the vitreous base is important in the development of peripheral retinal tears and rhegmatogenous detachments. The vitreous base normally extends from 2 to 4 mm posterior to the ora (Fig. 14). Within this region, collagen fibrils of the vitreous cortex insert into the internal limiting membrane. With aging, the posterior edge of the vitreous base migrates posteriorly and forms irregular excavations around the globe. The vitreoretinal adhesion is especially strong around this irregular posterior edge.25,26 The significance of this is that the posterior limits of the contour can exert great stress on the retina as the vitreous base retracts forward during aging, leading to a retinal tear at the posterior edge of this adhesion.
Histologic Features of the Ora Serrata
The histologic features characteristic of the ora serrata include thinning of the retina caused by a gradual loss of the nerve fiber layer, ganglion cell layer, and plexiform layers (Fig. 15). The outer nuclear layer is reduced to two or three cells in thickness and fused with the plexiform layers.14 This lack of neurons is compensated for by the neuroglia and Müller cells, which serve as the structural matrix for the entire retina. The internal limiting membrane is thickened in a 4-mm-wide zone at the ora because of the insertion of the vitreous base into this region. The rod photoreceptors disappear 1 mm posterior to the ora, where they are replaced by malformed cones. The external limiting membrane continues forward beyond the ora as the layer separating the pigmented from the nonpigmented ciliary epithelium. However, it has morphology different from that of the external limiting membrane of the retina.
A unique feature of the ora is that within its 2-mm width is the transition of the single-layered nonpigmented epithelium of the pars plana into the complex multilayered retina.27 The original light-microscopic observation of the ora suggested that this was a sudden change. Electron-microscopic studies have shown that the transition is gradual and is a recapitulation of retinal embryogenesis (see Fig. 15). In the most peripheral section of the ora, the retinal cells are primitive neuronal precursor cells. These undifferentiated retinal cells at the ora may serve as the storehouse for cells for retinal growth in eyes that grow during early postnatal life or in degenerative myopia. However, the appearance of maturation of the primitive retinal cells at the ora does not always indicate continuous retinal growth, because a similar structure of the ora is seen in eyes of all age groups. Posterior to these precursor cells, 0.28 mm posterior to the ora, undifferentiated photoreceptors are seen. These cells have neither an outer nor an inner segment. In addition, they lack the tight junction connections to the pigmented epithelium that are present between the nonpigmented epithelial cells of the pars plana and the pigmented ciliary epithelium. This explains why retinal detachment usually extends to the border of the ora serrata but only rarely more peripherally.
Farther posteriorly, the photoreceptors take on the configuration of those in the developing retina of a 23- to 36-week-old fetus. The inner segment consists of a mass of cytoplasm protruding past the external limiting membrane. Synaptic connections are occasionally discernible. Slightly more posteriorly, primitive outer segments can be seen. Although they lack the infolding of the plasma membrane characteristic of mature rod outer segments, some of the inner segments in cells have a cilium. Only slightly farther, at the posterior edge of the ora, the photoreceptors assume the adult form found throughout the rest of the retina. The remainder of the neurons make their appearance gradually as the photoreceptors become more mature.
Aging-Related Changes at the Ora Serrata
In an older eye, the histologic changes characteristic of the ora are seen extending more posteriorly than in a young eye. In addition, the ora serrata is subject to degenerative changes with aging. The inner limiting membrane becomes thickened and often vacuolated, and the retinal stroma shows cystoid spaces (Fig. 16). These cysts are usually aligned with dentate processes and are located just posterior to the ora serrata. These cysts may be in the outer plexiform layer (typical cystoid) or in the inner plexiform layer (reticular cystoid). They are formed within Müller cells and neuroglia and are filled with acid mucopolysaccharide. Occasionally, these cysts may communicate with the vitreous cavity or coalesce to develop retinoschisis.
The physiologic basis for the aging changes in the peripheral retina is unclear. One contributing factor may be the relative avascularity of the ora serrata. The retinal circulation stops approximately 1.5 mm behind the posterior limits of the ora bays, and the ora obtains its nourishment from the choroidal circulation.28 This relative avascularity may result in premature senescence of the retinal tissue of the ora, causing cystoid degeneration to occur.
RETINAL EMBRYOGENESIS
The eyeball starts to develop from neuroectoderm around the twenty-second day of fetal life. A pair of optic primordium, identified as optic pits, form on both sides of the midline in the ventrolateral region of the primitive forebrain. At approximately the 3-mm stage of development, the optic pits extend outward as hollow spheres ventrolaterally from the neural tube to form the optic vesicles.29,30 They become separated from the forebrain by a constriction, or stalk. The cavity of the future third ventricle is continuous with the cavity of the optic stalk and vesicles (Fig. 17A). The next event is the invagination of the optic vesicles, by differential growth and buckling, to form the optic cup. This occurs around the fourth week of fetal life. The optic cup forms a fold inferiorly and ventrally to form the “embryonic fissure” through which the mesenchymal and vascular tissues enter the globe (see Fig. 17A). The embryonic fissure begins to close midway in the fissure and extends anteriorly and posteriorly. The process is completed by the end of the seventh week of gestation. Incomplete closure of the fissure can result in colobomas of the iris, retina, or choroid.31
The retina develops from the neuroectoderm following the formation of the optic cup. The inner layer of the optic cup develops into the retina, and the outer layer develops into the retinal pigment epithelium. The differentiation of multipotent stem cells of the single-layer optic vesicle ectoderm into the multicellular mature retina is an ordered event that follows discrete stages. Recent studies indicate that cell fate determination in the vertebrate retina depend on changes in the response of the mitotic cells within the retina as well as changes in environment around these cells.32 The first stage is the differentiation of the cells of the optic vesicle into a two-layered tissue, the “neuroepithelium.” This stage of development commences simultaneously with the formation of the optic cup. During this process, a layer of ependymal cells that show numerous mitoses appears at the edge of the vesicle. At the base of these cells is a basement membrane from which extend numerous fine cilia.29 Interdigitation of the cilia with the cells of the outer layer of the optic vesicle is a precursor to the subsequent arrangement of the insertion of the photoreceptor outer segments into the spaces between the microvilli of the pigmented epithelium. Immediately internal to the ependymal layer is the thick layer of primitive undifferentiated cells, which are characterized by cytologic evidence of high mitotic and metabolic activity—large oval nuclei with darkly staining nucleoli and a prominent chromatin net. The ependymal layer and the aggregation of the nuclei of the undifferentiated cells form the “primitive zone,” constituting the outer 90% of the neuroepithelial thickness. The inner 10% is made up of the “marginal zone,” which consists of the cytoplasmic extensions of the multitudinous outer primitive cells. The marginal layer is microscopically differentiated by its lack of nuclei until the 10-mm stage at 6 weeks of development.
At the beginning of the invagination of the optic vesicle, the distal end of the primitive ophthalmic artery grows into the embryonic fissure from below. When the fissure closes, the artery becomes trapped within the cavity separating the marginal zone from the lens vesicle. Intraocular branches of this transitory hyaloid artery (Fig. 17B) presumably meet the nutrient requirements of the developing retina until its own vasculature is established during the fourth month of gestation.
From the sixth week to the third month, the neuroepithelium gradually develops into the neuroblastic layers, from which the mature retina subsequently develops. The pattern of this change resembles the morphogenesis of the central nervous system, with the spread of cellular proliferation directed from the inner layer toward the outer layers. Because of its invagination, the neuroepithelium’s direction of proliferation is translated into progression from the outer toward the inner layers (Fig. 17C). The primitive cells internal to the ependymal layer divide and migrate inward to form a distinct new layer of nuclei where the marginal layer had been (see Figs. 17B and 17C). This results in the formation of the inner and outer neuroblastic layers, which are separated by the transient nerve fiber layer of Chievitz (see Figs. 17B and 17D). This distinct, three-layered structure is temporary. It gradually takes on the more complex architecture of the retina by cellular differentiation. This differentiation of the neuroblastic layers progresses from the inner layer (future ganglion layer) to the outer layer (future photoreceptor layer) such that the outer retina takes on its mature appearance last.33 In addition, this differentiation of the neuroblastic layers is more precocious in the posterior pole as compared with the periphery (see Figs. 17B and 17E), and the development of the supportive structures occurs earlier than that of the neural structures.
Exemplifying these developmental principles is the finding that the Müller cells, which are the primary supportive elements of the retina, are the first of the retinal cells to appear, and they are found at the posterior pole as early as the 10-mm stage. The original position of the Müller cells is adjacent to the internal limiting membrane, which they contact with their inner processes. This results in a double-layered internal limiting membrane whose inner component is the basement membrane formed by the original neuroectodermal cells, and the outer layer is the pseudomembrane formed by the adhesion of the terminal processes of the Müller cells. The Müller cells elongate to extend through the full thickness of the retina until they insert onto the outer basement membrane, which was previously formed by the neuroectodermal cells. The external limiting membrane forms when the Müller cells develop junctions with their contiguous neighbors. Emerging evidence indicates that the molecular basis for regulating Muller cell development may depend on intracellular and extracellular signaling pathways. The Notch, a contact-mediated signaling pathway, as well as cyclin dependent kinase inhibitors have been implicated to play a role.34
Once Müller cell differentiation occurs, the third stage of retinal development follows with the differentiation of the neural elements. The inner neuroblastic layer undergoes this differentiating process before the outer layer does (Fig. 18A and 18B, top). The ganglion cells migrate inward from the inner neuroblastic layer, creating a gap between them and the remainder of the inner plexiform layer. The nerve fiber layer becomes the first mature retinal layer;35 it gradually forms from the 17-mm (see Fig. 17D) to the 130-mm stage as the ganglion cells send their axons toward the optic disc.
The next step in differentiation occurs between the 17-mm stage and the fourth and fifth months of development. The inner nuclear layer is created by inward migration of a part of the outer neuroblastic layer and joining of these cells with the amacrine and müllerian cells of the inner neuroblastic layer (see Fig. 18A and 18B, bottom). This results in the obliteration of the layer of Chievitz and the formation of the outer plexiform layer, which occur during the 10th and 12th weeks of gestation. These developmental changes occur from the posterior pole to the periphery of the retina, with the exception of the macula, which is the last region to undergo development.36 The remainder of the outer neuroblastic layer cells, which are precursors to photoreceptor cells, become a row of cells with the nuclei internal to the outer limiting membrane by the fifth to sixth month. This establishes the retinal layers that will persist into maturity. However, the morphologic development into primitive photoreceptor cells does not begin until the 48-mm stage (see Fig. 18A and 18B, bottom).
The photoreceptor cell bodies derive from these late-developing outer neuroblastic cells, and the photoreceptor outer segments arise from cytoplasmic processes that either originate from or replace the cilia of the original outer membrane at the 17-mm stage.29 These processes rapidly form interdigitations with the microvilli of the pigmented epithelial cells. At a far later time, at approximately the 80-mm stage, cells of the outer nuclear layer extend protoplasmic processes that penetrate the original external basement membrane and form the inner portion of the photoreceptor outer segments. Thus, the photoreceptor outer segments form developmentally as a separate unit and become connected to the remainder of the photoreceptor cell by a narrow stem later in embryogenesis.
The genesis of retinal architecture follows a spatiotemporal gradient probably regulated by local interactions.37,38 Action potentials endogenously generated by the ganglion cells, the first cells to differentiate in the neural retina, appear to be necessary for development of the retinal layers.39 Spontaneously generated calcium waves have been detected in ganglion and amacrine cells of the immature retina before photoreceptor development. These waves are synchronized and spread horizontally in a wavelike manner across the retina.40 They are hypothesized to refine the neural connections and confer topographical mapping to retinal connections. The temporal characteristics of these calcium waves appear to be regulated by GABA, a neurotransmitter expressed by human fetal retina as early as 12 weeks of gestation.41,42
The formation of the vascular system in the internal retina by the eighth month marks the end of retinal maturation, except for foveal development, which continues until 6 months after birth. Any growth of the retinal area beyond its size at the eighth month of fetal development results from thinning of the existing structure, because there is no further mitotic proliferation or cellular hypertrophy of retinal neurons after that time. This accounts for the peripheral retinal thinning characteristic of myopic eyes in which globe enlargement occurs years after completion of the tissue’s growth potential. Similarly, the normal retina cannot regenerate after destruction of retinal tissue by infection, inflammation, vascular insufficiency, surgical photocoagulation, diathermy, or cryotherapy. In these cases, retinal replacement occurs only through glial cell metaplasia and hyperplasia.
The macula is the last retinal region to reach morphologic maturity.29,30 The macula retains the transient layer of Chievitz until 4 months after birth. The ganglion cells continue to accumulate in the macula until the sixth month of fetal life, with no evidence of the tissue thinning that characterizes the growth of all other retinal regions. Studies in monkeys indicate that the formation of the foveola and the development of the morphology of cells within the foveola depend on the formation of high densities of retinal ganglion cells within the central retina.43 The foveal pit results from the gradual loss of overlying ganglion cells as they spread slightly peripherally during months 7 and 8. Similarly, the amacrine, horizontal, Müller, and bipolar cell nuclei overlying the center of the fovea are displaced.44 Until 4 months after birth, the macula continues to mature with elongation and full development of the cones and peripheral migration of the remaining ganglion and bipolar cells. The result is the disappearance of the layer of Chievitz and the formation of the foveal pit. This late maturation of the fovea accounts for the delay in development of fixational ability until the postnatal age of 4 months.
MORPHOLOGY OF RETINAL NEURONS
The cells within the retina can be divided into neuronal, glial, and vascular components. The neuronal cells are responsible for the primary function of the retina (i.e., conversion and transmission of incoming light into an electrical signal perceived by the brain). This is achieved through intricate synaptic connections among three different classes of retinal neurons, namely, photoreceptors, interneurons, and ganglion cells. A detailed understanding of the cellular morphology of these neuronal cells and their synaptic connections has been possible in recent years with the advent of electron microscopy, immunohistochemistry, and Golgi-impregnated preparations. Morphologically, 55 different types of retinal neurons have been identified in the mammalian retina to date. The function of 22 of these cell types is known. Each morphological type of retinal neuron appears to have a distinct physiological function. As further studies improve our understanding of the retina’s role in processing the visual signal, the function of the remaining identified cell types will become apparent.
GENERAL STRUCTURE OF THE SYNAPSE
To appreciate the complexity of the interneuronal connections within the retina, an understanding of the basic morphology and function of synapses and other interneuronal junctions is important. The retinal neurons form three major types of intercellular connections: the chemical synapse for neuronal signal transmission, the zonula adherens that maintains tissue structure, and the gap junction that provides electrical transmission between developing retinal cells. These junctions have important morphologic similarities and differences that reflect their differences in function.
The synaptic junction of a typical chemical synapse consists of specialized apposed membranes of the two neuronal cells, separated by a 20- to 30-mm cleft.45 Similar to other intercellular junctions, such as zonulae adherentes, the plasma membrane of the synaptic junction contains tufts of fine filaments that insert into cytoplasmic densities, and the two apposing cell membranes are glued together by a thin, dark plate of filamentous material in the intercellular cleft.46 However, unlike the interepithelial cell adherence, the interneuronal synapse is polarized and has an asymmetric predominance of intracytoplasmic fibrils in the postsynaptic side of the junction.
The distinction between the morphology of the synaptic junction and that of the zonula adherens is important because both types of junctions are present between neuronal cells in the retina. These nonsynaptic junctions do not serve the purpose of electrical communication between cells. As in epithelial tissue, where they are commonly found, the zonulae adherentes act as binding sites to maintain tissue cohesion.45 In neural tissue they are usually short and have been given the special label puncta adhaerentia.46
Another type of junction between neuronal cells in the retina is the gap junction.47 In a gap junction, there is close apposition between presynaptic and postsynaptic membranes, and current is allowed to pass directly between adjacent cells. Thus, it is classified as an electrical synapse. Because no chemical mediator substance is involved, the rate of signal transmission is faster in these junctions than in typical chemical synapses. In some synapses, characteristics of both the chemical synapse and the gap junction are found. These connections, called “mixed synapses,” have not been found in the retina thus far.45
The electrical synapse (gap junction) characteristically shows a transsynaptic gap with a seven-layered structure produced by the approximation of membranes from the two juxtaposed cells (Fig. 19).48 The synapse consists of four dense lines and three intervening light spaces. It lacks the morphologic detail characteristic of the chemical synapse. Thus, they are localized best by tracer coupling. There are no vesicles and no obvious changes in the cytoplasm on the two sides of the junction. The gap junction does not function by the use of a chemical transmitter. Instead, it offers a low-resistance pathway between the cells. The extent of the electrical coupling of the adjacent cells appears to be dependent on the level of expression and type of the connecting particles (connexin). Thus, plasticity of the coupling in the retina is possible and the retina has the potential to optimize vision for the ambient lighting conditions.49
![]() Fig. 19 Electron micrograph of a gap junction between two horizontal cells in a teleost retina. (Courtesy of Dr. Charles Zucker) |
The close apposition of the plasma membranes of the gap junction allows for rapid and well-insulated electrical transmission to occur in either direction. Gap junctions have been identified between photoreceptor terminals, horizontal cells, and amacrine cells.50,51,52,53,54 In the vertebrate retina, gap junctions usually mediate lateral connections, coupling neurons of a single subtype into an extended array of mosaics in the plane of the retina (homologous).55 Occasionally, these electrical synapses can be seen between bipolar terminals and amacrine cells processes (heterologous).56,57 In these instances, they may play a role in transmitting signals from the photoreceptor to the ganglion cell.58 Based on biotinylated tracer studies, at least 3 different types of gap junctions have been found.59 The most permeable gap junctions were between A-type horizontal cells. The lowest permeability gap junctions were found between cone bipolar cells and AII amacrine cells. Intermediate range gap junctions were found between amacrine cells and between B-type horizontal cells.
In the retina, the major type of interneuronal junction of interest is the chemical synapse. In such a synapse, intercellular communication is achieved when a chemical substance, the neurotransmitter, synthesized from the presynaptic axon terminal, is released into the synaptic cleft, where it binds to specific receptors on the postsynaptic membrane. This binding of the neurotrans-mitter elicits an electrophysiologic change in the postsynaptic neuron that may be excitatory or inhibitory.60 This response, in turn, can be affected by neuromodulators released by neurons that can alter the metabolism of the neurotransmitter or the postsynaptic electrophysiologic response. Table 1 summarizes the putative neurotransmitters and neuromodulators that have been associated with presynaptic terminals of various retinal neurons.60,61,62 Immunohistochemical studies show that each of these neurotransmitters may be associated with only a subpopulation of a type of retinal neuron.63 In addition, there may be co-localization of more than one neurotransmitter in the same retinal neuron.62,64 In photoreceptors, only glutamate and aspartate have been identified as neurotransmitters. Colocalization of four or more neurotransmitters is often observed in the interneurons.64,65
TABLE 1. Putative Neurotransmitters and Neuromodulators of the Retinal Neurons | |||||||||||||||||||||||||||||||||||||||||||||||||||||||||||
---|---|---|---|---|---|---|---|---|---|---|---|---|---|---|---|---|---|---|---|---|---|---|---|---|---|---|---|---|---|---|---|---|---|---|---|---|---|---|---|---|---|---|---|---|---|---|---|---|---|---|---|---|---|---|---|---|---|---|---|
|
The basic feature of the presynaptic elements of the chemical synapse is the boutons terminaux at the ends of the axons, or boutons en passant along their length.45 In the central nervous system, these occur at points where myelination has ceased. The lack of myelination in the retina makes it impossible to use myelination gaps for synapse identification. Although most synapses are axodendritic, axosomatic, or axoaxonal, other types have been documented in the brain and the retina where the presynaptic component is not axonal but dendritic or somatic. However, all of these synaptic types share a common morphology consisting of presynaptic and postsynaptic membranes, the densities associated with their cytoplasmic faces, and the synaptic cleft between them.
In the retina, three different classes of chemical synapses have been described based on the appearance of the presynaptic element: conventional synapse, ribbon synapse, and flat or basal junction.66 The conventional synapse, which is similar in morphology to other chemical synapses found throughout the vertebrate nervous system, has aggregates of synaptic vesicles clustered close to the presynaptic membrane. These vesicles are membrane-bound organelles that contain neurotransmitter substances and enzymes involved in neurotransmitter metabolism.67 They lie in the midst of a hexagonal array of electron-dense particles, each 30- to 50-nm wide and separated from neighboring particles by an interval of 20 to 50 nm.68 This electron-dense material is believed to play a role in the attachment of the synaptic vesicles to the presynaptic membrane. These synapses are found in the presynaptic terminals of horizontal cells, amacrine cells, and interplexiform cells.66 They typically have an inhibitory effect in the postsynaptic neuron.
The ribbon or invaginating synapse, in contrast, is usually found in the presynaptic terminals of photoreceptors and bipolar cells in the retina (Fig. 20). They are also often observed throughout the invertebrate nervous system. The presynaptic terminal of the ribbon synapse is characterized by the presence of an electron-dense bar or a ribbon of approximately 1 um length that is oriented perpendicular to the presynaptic membrane. Reconstruction reveals that this ribbon is in fact a rectangular or horseshoe shaped plate composed of two osmiophilic electron-dense lamellae flanking an electron-dense central layer69,70 This synaptic ribbon is surrounded by a precisely arranged array of synaptic vesicles and appears to be anchored to the presynaptic membrane by three to five delicate electron-dense filaments. The shape and size of the synaptic ribbons can change depending on the maturation stage, activity state of the synapse, or light/dark cycle. Although the precise function of the synaptic ribbon is unknown, it has been hypothesized to function as a “conveyor belt” to channel synaptic vesicles to the presynaptic membrane for neurotransmitter release, to immobilize the vesicles in inactive synapses, storage device for synaptic transmitter, and to act as a diffusion barrier for presynaptic ions.69 In the inner plexiform layer, this synapse has two postsynaptic processes, forming a “dyad.” In the outer plexiform layer, there are three postsynaptic processes, forming a “triad” (see Fig. 20).
Basal junctions are found in the presynaptic terminal of photoreceptors only.66 In the mammalian retina, these junctions are found only in association with cone cells. Typically, the presynaptic membrane is smoothly indented, and there is prominent electron-dense material on the inner surface of the terminal membrane. However, no synaptic vesicle is seen in association with the electron-dense material. The exact mechanism for chemical transmission through these synapses is unclear. However, in axonal terminals of goldfish horizontal cells in which no presynaptic vesicles are found, neurotransmitter release is believed to occur by a carrier-mediated mechanism.71 Such a process may be involved in basal junctions of mammalian photoreceptors. The involvement of a neurotransmitter in signal transduction is implicated by freeze-fracture studies that show that the postsynaptic membranes of these synapses contain particles that are similar to those found in the postsynaptic membranes of these synapses contain particles that are similar to those found in the postsynaptic membranes of a conventional synapse in the brain.
The synaptic cleft of a typical chemical synapse is 20- to 30-nm wide.45 It is not a free space because it houses material that binds the presynaptic and postsynaptic membranes together in a strong adhesion. The electron-dense plaque in the middle of the synaptic space contains mucopolysaccharides and proteins, which may serve to maintain the attachment between the presynaptic and postsynaptic membranes through a form of polyionic binding. Filaments composed of macromolecules span the synaptic cleft. This synaptic cleft substance may be important for formation, localization, and proper function of the synapse. Macromolecules spanning the gap between the presynaptic and postsynaptic membranes may ensure rapid transport of the transmitter molecule through the cleft and may prevent the transmitter from diffusing away from the synapse.72
In the postsynaptic membrane, a linear density is present. From this structure, filaments project out toward the cytoplasm.45 These densities form either a single large or several small discs, which may be interspersed with puncta adhaerentia. The anatomic location of the receptors for the transmitter molecule in the postsynaptic membrane has not yet been determined. However, freeze-fracture studies have shown minute bumps within the specialized zone of the postsynaptic membrane that may represent the neurotransmitter receptor molecule.66 In the case of the neuromuscular junction, alpha-bungarotoxins that bind to the acetylcholine receptor have been localized in the regions of the postsynaptic membrane. It presently is believed that the specialized region of the postsynaptic membrane contains the receptor-ionophore complex responsible for the postsynaptic changes in ionic permeability.73 Consistent with this hypothesis, electrophysiologic studies have shown that there is a sharp, focal increase in sensitivity to the transmitter in the postsynaptic membrane bouton, suggesting that the receptors for the transmitter are present in high density in this region.74 However, this postsynaptic specialization may be reversible, because this localized distribution of transmitter receptor activity is lost and receptor activity is detected in the extrasynaptic membranes of the postsynaptic cells when presynaptic neuronal input is inhibited.
Extensive research has been done recently to identify receptors for the neurotransmitters in the postsynaptic neurons of the retina. The neurotransmitter receptors are extremely diverse in their subunit composition and their exact actions and the signaling pathways on the postsynaptic neurons are not fully elucidated. Glutamate receptors have been identified on bipolar, horizontal and amacrine cells.75 Glycine receptors have been identified on ganglion and bipolar cells.76,77,78 GABA receptors have been found on photoreceptors, bipolar cells and ganglion cells.78,79,80 Nicotinic acetylcholine receptors have been found on amacrine and ganglion cells.81 Purinergic receptors have been found on bipolar cells.82 Dopamine and somatostatin receptors are found on all retinal neurons.65,83 However, most of the dopaminergic actions appear to be extrasynaptic. Further studies are needed to complete our understanding of the role of these neurotransmitters in the visual signal processing of the retina.
PHOTORECEPTOR CELLS
The photoreceptor cells of the retina consist of the rods and cones. These are the primary neurons in the visual pathway. Because they derive embryonically from the ciliated cells lining the optic vesicle cavity, they come to lie at the outer edge of the retina. The dense packing of the photoreceptors, as well as their precisely axial arrangement relative to the optical system of the ocular media, provides for detailed sampling of the visual field. Any change in the arrangement of these long, narrow cells so that they are no longer axial with the impinging light results in alteration in vision. Micropsia results if the photoreceptors are abnormally separated from each other but maintain their normal alignment axial to the optical system. This can occur with central serous retinopathy. Metamorphopsia results if the alignment is partially lacking, as in cellophane maculopathy or serous elevation of the macula. Loss of visual acuity can result if the alignment is sufficiently disturbed so that the photoreceptors are no longer relatively axial to the impinging light and lose their sensitivity.
The photoreceptor cells differentiate longitudinally into four major regions: the inner segment containing the metabolic apparatus, the outer segment containing the visual pigment for the conversion of light into neuroelectrical energy, a perikaryal region containing the cell nucleus, and a synaptic terminal.11 The inner and outer segments of both rods and cones are connected by a thin cytoplasmic bridge containing a cilium (Fig. 21).
Histologically, the photoreceptors are classified into two types: rods and cones. They differ in the shape of the inner and outer segments, position of the nucleus, and shape of the synaptic terminals (see Fig. 21). The outer segments in rods and foveal cones are cylindrical, whereas those in extrafoveal cones are tapered.11 In general, a cell with the cone-like outer segment is associated with a large synaptic terminal, called a pedicle. The synaptic terminals of rods are smaller and are called spherules (see Fig. 20).
In addition, rods and cones have a different topographic distribution in the retina. Although the rods far outnumber the cones in the total retina, with a ratio of 125 million rods to 6.5 million cones, the cones are present predominantly in the foveal region, where they reach a density of 150,000 per square millimeter.12 The cone density decreases to 75,000 per square millimeter at 130 μm from the foveal center, at which point the rods begin to appear. Approximately 3 mm from the foveal center, the rods are present at their highest density, whereas the density of the cones continues to diminish.
Retinal photoreceptor density decreases with increasing age in humans. The annual cell loss is approximately 0.2% to 0.4%, similar to age-related loss of retinal ganglion cells and retinal pigment epithelial cells.84 Rods are more affected than cones. The decline in density is more pronounced 5 to 8 mm from the foveal center than the retinal periphery. All quadrants of the retina appear to be equally affected. This decline may play a role in age-related retinal disorders. In patients with age-related macular degeneration, an even more pronounced loss of the rods are noted in the parafovea when compared to age-related controls.85 Recent immunohistochemical studies of postmortem eyes indicate increased apoptosis in the macular photoreceptors in patients with atrophic macular degeneration (Fig. 22).86 In patients with advanced macular degeneration, virtually all surviving photoreceptors in the macula are cones, a reverse of the normal macula which contains mostly rods.85 Similarly, among patients with retinitis pigmentosa, mutations in proteins of the photoreceptor outer segments appear to selectively cause apoptosis of rods. Cones do not appear to be directly affected by the mutation but appear to die secondary to rod cell death. The mechanism of cone cell death in retinal degeneration is unknown at present.
Outer Segments
The outer segments of rods and cones differ in their size, shape, and structure. Rod outer segments are 25- to 28-μm-long cylindrical structures with a diameter of 1 to 1.5 μm. The outer segments of extrafoveal cones are shorter and tapered—the diameter decreases from 6 μm at the base to 1.5 μm at the tip. The outer segments of foveal cones are tightly packed and elongated and appear more like rod outer segments.87,88
Both rod and cone outer segments contain an elaborate system of stacked membranous discs that arise from invaginations of the cell membrane during development. The main morphologic difference between the rod and cone outer segments is that these discs are continuous with the cellular membrane of the outer segments in cones, whereas in rods they have no continuity with the surrounding cell membrane except at the base of the outer segment.89 Despite this “free-floating” appearance of the rod outer segment discs, they are held in place by a filamentous cytoskeletal structure that spans the rims of these discs.90
The membrane of the outer segment discs contains 90% of the photopigment.11 Each outer segment disc of rods contains 300 to 900 molecules of the photopigment.90 In rods, the visual pigment called rhodopsin is composed of the light-absorbing chromophore retinal, which is attached to a protein moiety called opsin.91 This photopigment is most sensitive to light that is approximately 500 nm in wavelength. Similar photopigment has been found in cones. However, there are three different types of cones, and each cone type contains a different protein moiety in the photopigment. This results in three different spectral sensitivities among the cone photopigments. The blue-sensitive cone pigment has maximal absorption of light at approximately a 450-nm wavelength, the green-sensitive cone absorbs at approximately 530 nm, and the yellow-sensitive cone absorbs at approximately 565 nm. Defects in color vision result from absence or dysfunction of one or more of these cone types.
During the 1960s, Young showed evidence that the rod outer segments are constantly renewed.92 Subsequently, it has been shown that cones also shed their discs.93 In rods, the sacs are formed in the inner segment of the photoreceptor cell and shed at the outer segment tip. These shed sacs, in turn, are phagocytosed by the adjacent pigment epithelial cell (Fig. 23). This cycle of disc shedding has been found to be a circadian phenomenon in some species. In others, it appears to be triggered by light. The physiologic factors that regulate the cycle of disc shedding are still unknown and under investigation.
The formation of photoreceptor discs in rods begins with the synthesis of rhodopsin in the rough endoplasmic reticulum and Golgi apparatus of the inner segment.94 Here, the protein is inserted into the lipid bilayer of the endoplasmic reticulum, which eventually fuses with the apical inner segment plasma membrane. The protein is thought to diffuse in the plane of the cell membrane and become incorporated into new disc membranes formed at the base of the outer segment.
When the sacs first appear in the base of the outer segments, they are immature, with poorly oriented discs and membranous tubules. As these sacs increase in number and size, they move distally and take on a mature appearance as they become oriented parallel to the older sacs. The entire progression down the outer segment requires approximately 9 to 13 days. New discs are formed at the rate of approximately 90 per day.95 When the sacs reach the distal end of the rod outer segment, their tips detach and undergo phagocytosis by the adjacent pigment epithelial cell. Recent evidence indicates that a disturbance of the pigment epithelial cell’s phagocytic capacity may be a factor in the retinitis pigmentosa class of retinal degenerations.93 Whether this is caused by a primary malfunction of the pigment epithelial cell or to an abnormality of the outer segment that prevents normal phagocytosis is unknown. In studies of dystrophic rat retinas where there is a defect in phagocytosis, the primary defect in gene expression appears to be at the level of the retinal pigment epithelium.96
The discs of cones have a pattern of growth different from that of rods. Radioactive precursor studies show that new protein is incorporated into the cell membrane throughout the outer segment, rather than just at the base.97,98 This implies that cone outer segment renewal is a constant process that is under way at many points throughout the outer segment. This correlates with the previously mentioned structural difference between the rods and cones, wherein cone discs are actually invaginations of the cell membrane, and the rod discs appear to be completely separate from the cell membrane except at the base.
The photoreceptor outer segments have two important connections. One of these is to the inner segment, which is actually the cell body of the photoreceptor (see Fig. 21). The other connection is to the extracellular matrix that separates the photoreceptor outer segment from the pigment epithelial cell. Radioactive tracer experiments suggest that the sources of this extracellular matrix substance are the pigment epithelial and photoreceptor cells.99,100 The material, a glycoprotein consisting of chondroitin sulfate and sialic acid, is secreted into the space between the outer segments and the pigment epithelial cells by vesicles that originate in both cells. The function of this mucopolysaccharide-rich matrix is not well understood. It may provide a major route by which metabolites and nutrients pass between photoreceptor cells and their vascular supply. It may constitute the only intercellular bond between the outer segments of photoreceptors and the pigment epithelial cells, because there are no cellular connections such as tight junctions between these cells (see Fig. 23). Recent studies have identified interphotoreceptor retinol-binding protein as a major component of this matrix.100 This glycoprotein is believed to mediate the transport of a vitamin A derivative, retinol, between the photoreceptor and the pigment epithelium.
The connection of the outer to the inner segment of the photoreceptor is through a slender (0.2–0.3 μm in diameter) neck that is eccentric toward one side of the cell (see Fig. 21). The cilium, which is the embryonic basis for origin of the photoreceptors, is located in the neck and extends into the basal one third of the outer segment. It has nine pairs of microtubules. However, unlike motile cilia, the cilium has no microtubules centrally. The microtubules end in a modified centriole in the apex of the inner segment. The cilium functions as a conduit for metabolic materials going from the inner to the outer segments.
Inner Segments
The inner segment of the photoreceptor is the portion of the cell that metabolically services the outer segment. Whereas the outer segment shows high differentiation, containing only equipment necessary for the photoreceptor process, the inner segment possesses the cellular machinery essential for the metabolic and synthetic functions of the cell. There are two distinct morphologic regions in the inner segment, the ellipsoid and the myoid. The most prominent feature of the outer portion, or ellipsoid, of the inner segment is the abundance of large mitochondria at the apex (see Fig. 21). The ellipsoid appears to be more sensitive to anoxia than any other part of the photoreceptor cell.101 The staining characteristics of this area vary with the state of metabolic activity of the photoreceptor cell and may account for the subtle staining differences seen in the various types of photoreceptor cells. The mitochondria in the ellipsoid are compactly arranged. They are present in higher concentration in retinas with poor vasculature. In addition, the cones have a higher concentration and greater absolute number of mitochondria than the rods. There may be up to 600 mitochondria per cone.87 These mitochondria contain the normal enzymes for oxidative production of energy.102
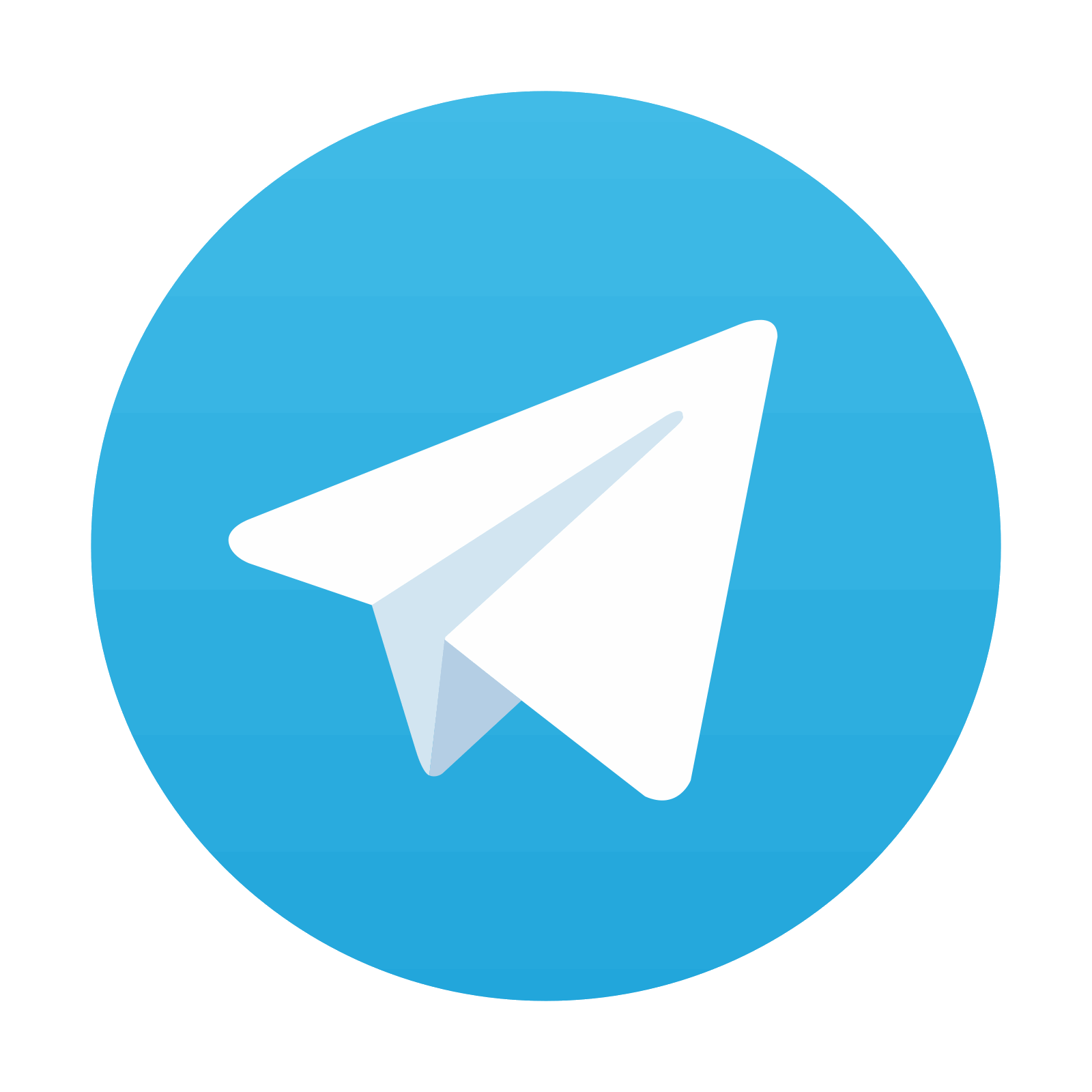
Stay updated, free articles. Join our Telegram channel
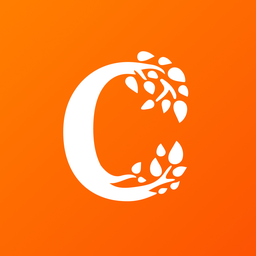
Full access? Get Clinical Tree
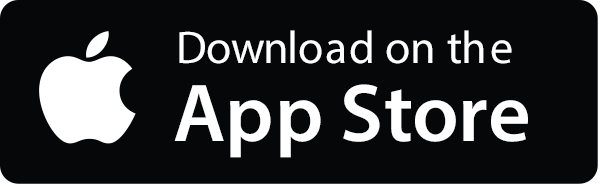
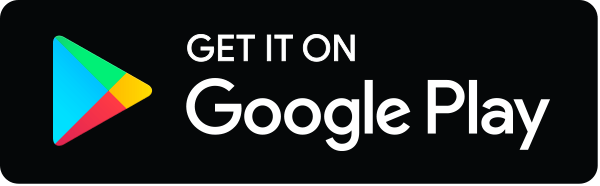
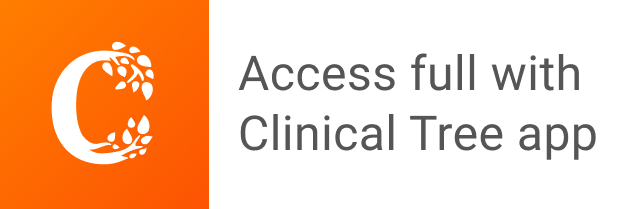