![]() FIGURE 1-1. Diagram demonstrating the ocular surface and adnexal tissues described in this chapter. The ocular surface tissues include the cornea, limbus, and conjunctiva; and the adnexa include the lids with eyelash cilia, the meibomian gland, the main lacrimal gland, the accessory lacrimal glands of Krause and Wolfring and the small glands, that empty into the cilia sheath—the glands of Zeiss and Moll.(see color image) |
potential danger. In addition to its unique functions over the translucent cornea, the epithelium carries out “routine” housekeeping functions common to any epithelium that borders the external environment. These include provision of a barrier to fluid loss and pathogen entrance, and resistance to abrasive pressure. A barrier to fluid loss is provided by tight junctions surrounding lateral membranes of apical cells, and a pathogen barrier is provided by membrane-spanning mucins at the apical surface. Resistance to abrasion requires that cells of the epithelium be specialized for tight adherence to one another and to their underlying extracellular matrix. Finally, its position adjacent to the outside world requires that the epithelium have a rapid and highly developed ability to respond to wounding.
TABLE 1-1. CORNEAL EXTRACELLULAR MATRIX COMPONENTS | ||||||||||||||||||||||||||||||||||||||||||||||||||||||||
---|---|---|---|---|---|---|---|---|---|---|---|---|---|---|---|---|---|---|---|---|---|---|---|---|---|---|---|---|---|---|---|---|---|---|---|---|---|---|---|---|---|---|---|---|---|---|---|---|---|---|---|---|---|---|---|---|
|
glycoproteins. However, any glycoprotein with a collagenous domain is generally referred to as collagen. The remaining noncollagenous proteins in the stroma are subdivided into two major groups, glycoproteins and proteoglycans. Proteoglycans are a special class of glycoproteins that have glycosaminoglycan (GAG) side chains.
TABLE 1-2. COLLAGENS PRESENT IN CORNEA | ||||||||||||||||||||||||||
---|---|---|---|---|---|---|---|---|---|---|---|---|---|---|---|---|---|---|---|---|---|---|---|---|---|---|
|
proteins of lumican and decorin bind to collagen and limit the size of the fibril. The importance of proteoglycans in corneal stromal fibril formation is demonstrated in mice lacking lumican, which have corneal opacities as a result of irregular fibril size and spacing (64), and also in humans who have mutations in keratocan. This mutation results in corneal flattening (65).
synthesized by the epithelium in response to a wound [reviewed in Zieske (71)] (Table 1-1). These include laminin-5, entactin, collagen IV, and perlecan, which are normally present in the basement membrane. In addition, components not normally present are synthesized and deposited, including lumican, fibrin, and an unprocessed form of laminin-5. These components appear to be present in all types of wounds and make up a wound-healing surface that has, in the past, been termed a pseudomembrane, but is more properly termed a provisional matrix. Epithelial cells actually migrate on this provisional matrix after wounding, and several lines of evidence in animal models suggest that the matrix influences wound healing [reviewed in Zieske (71)]. For example, corneal epithelial cells in culture migrate at a faster rate on unprocessed laminin-5 than on the processed form seen in unwounded corneas. Stromal wound healing also appears to involve a provisional matrix. A number of extracellular matrix components not normally seen in the stroma have been localized after wounding, including fibronectin, fibrin, tenascin, collagen types IV and VII, and laminin-1 (Table 1-1). In addition, keratan sulfate proteoglycan levels decrease whereas chondroitin sulfate levels increase in the wounded stroma. In an experimental model, fibronectin and chondroitin sulfate stimulate fibroblast migration into a matrix. Thus, the provisional matrix may promote migration of fibroblasts into the wound area.
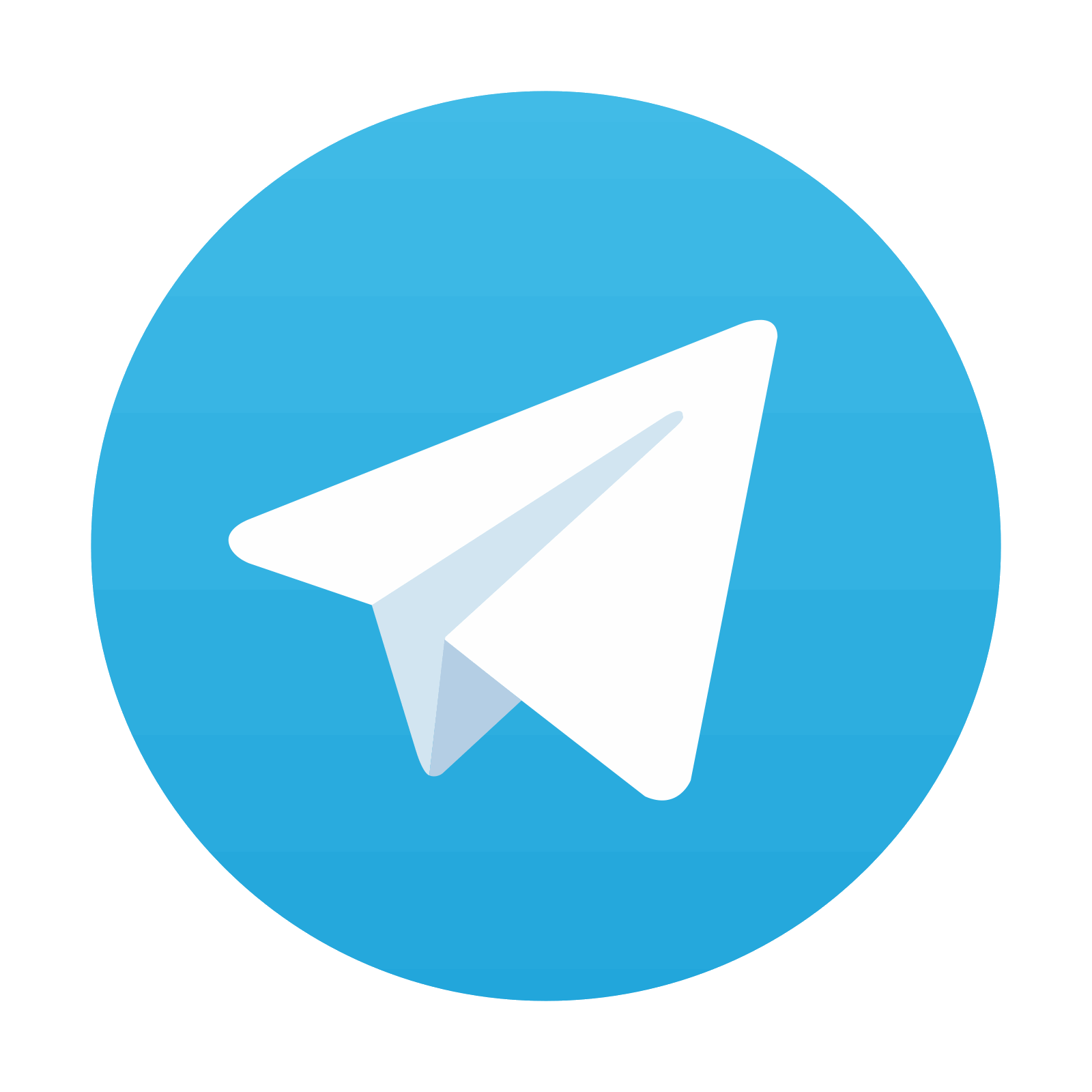
Stay updated, free articles. Join our Telegram channel
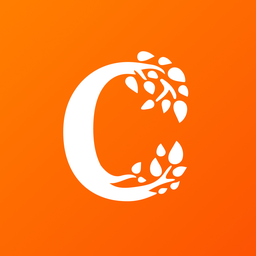
Full access? Get Clinical Tree
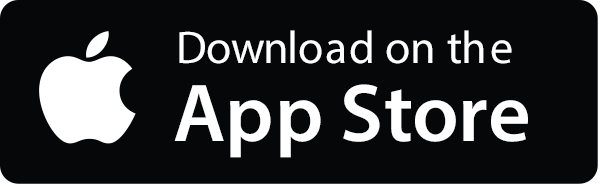
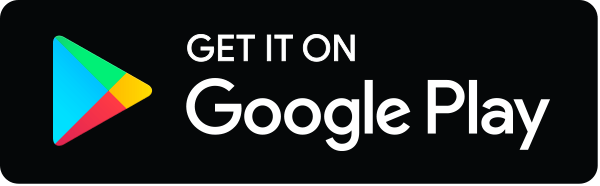
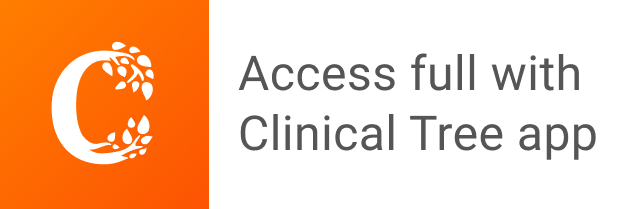