2 The Advent of the Femtosecond Laser in Medicine and Ophthalmology
Summary
Lasers were used initially within ophthalmology in the 1960s to perform retinal photocoagulation. The advent of newer and more sophisticated modalities capable of delivering shorter and more precise pulses later allowed ophthalmologists to treat various types of glaucoma and refractive diseases. The femtosecond laser (FSL), first introduced to ophthalmology in the 1990s, is an incredibly promising development whose potential warrants further exploration. Mode-locking studies during the 1960s and early 1970s, investigations into chirped pulse amplification during the 1980s, the development of the Ti:sapphire laser, and the discovery of Kerr-lens mode-locking in the late 1980s, all culminated in the production of the first FSL. This laser applies a highly focused, pulsatile laser beam to a target tissue, generating plasma consisting of free electrons and ionized molecules that rapidly expand and collapse, resulting in microcavitations and acoustic shock waves that separate and incise target tissue. The FSL employs ultrafast pulse times of 10–15 s, allowing for smaller amounts of energy to be used while maintaining similar power output, thus sparing delicate, adjacent tissues from collateral damage. Although first applied to laser-assisted in-situ keratomileusis (LASIK) in the early 2000s, the FSL has been used in cataract surgery, and multiple investigations in the past decade have shown that extension of the FSL to glaucoma and retinal procedures is promising. The FSL has also been applied to multiple medical domains as described briefly herein. In this chapter, we discuss how the FSL evolved and enhanced the treatment of ophthalmic disease.
Keywords: femtosecond, laser, IntraLase, LensAR, VICTUS, LenSx, Catalys, FEMTEC, Visumax
2.1 Introduction
Lasers occupy a unique domain within ophthalmology, specifically, and within medicine, in general, given their applications to diagnostics and to treating a variety of diseases and disease states. Historically, lasers were first used in an ophthalmic setting more than 50 years ago to create retinal lesions in rabbit eyes, paving the way for researchers to elucidate the principles of retinal photocoagulation—which is still used today to treat a host of maculoretinal pathologies. Through years of trial and error, the applications of laser technology expanded immensely. Nowadays, lasers play a significant role in treatment plans across a variety of ophthalmic disciplines. When viewed historically, one may argue that the narratives of laser development and of progress within ophthalmology are inseparable and delightfully synergistic. From the ruby laser, first constructed in the 1960s, to our youngest jewel—the femtosecond laser (FSL)—it is clear that laser advancements led to innovative techniques for treating eye diseases, and that new insights into eye pathologies led to improvements to existing laser technologies. The story of how the FSL came into being illustrates this point quite well, particularly when juxtaposed against the larger backdrop of how, throughout history, humans have attempted to harness (or more recently, to create) light in order to exploit its therapeutic benefits. In this chapter, we discuss how the FSL was later introduced in medicine and ophthalmology, and how it evolved and enhanced the treatment of ophthalmic disease.
2.2 Basic Concepts
It is worth revisiting in simple terms how a laser works. Light amplification by stimulated emission of radiation, or LASER for short, describes any device that creates and amplifies a narrow, focused beam of light. The setup of a laser includes a gain medium—usually either a crystal (solid state), such as ruby, or a gas or liquid—inside a highly reflective optical cavity consisting of two mirrors (one of which is partially transparent), as well as a light source to “optically pump” or excite atoms into higher-energy quantum states. Light is reflected off the mirrors and bounces back and forth within the medium such that the atoms or molecules of the medium attain a higher-energy state, which is also termed “population inversion.” Photons emitted during the decay of atoms in the excited state bounce back and forth and stimulate identical photons to do the same, creating a sudden burst of coherent light as the atoms discharge in a rapid chain reaction. The light then exits the medium through the partially transparent mirror and projects onto a target. Although initially used to treat retinal lesions in the 1960s, lasers eventually transcended multiple ophthalmic disciplines and found use in refractive, cataract, and glaucoma surgery in the 1970s until now. 1
2.3 The Development of the Femtosecond Laser
The FSL development began with several mode-locking studies within the theoretical and experimental physics community during the 1960s and early 1970s. Additional investigations into chirped pulse amplification (CPA) during the 1980s, as well as the development of the Ti:sapphire laser and the discovery of Kerr-lens mode-locking in the late 1980s, culminated in the production of a laser capable of operating in the femtosecond regime.
Laser mode-locking was identified in papers written by DiDomenico and Hargrove et al in the 1960s. 2, 3 While mode-locking is technically complex and a description of its quantum mechanical underpinnings is beyond the scope of this writing, the concept, in brief, is based on the fact that laser light is not a single, pure frequency or wavelength. Instead, laser light is emitted over a bandwidth or range of frequencies, determined primarily by the composition of the gain medium. If each laser mode operates with a fixed phase between it and the other modes, periodically the modes will interfere constructively with one another and produce an intense pulse of light. A greater number of modes oscillating in phase with each other shorten the pulse duration. Extending this principle to laser systems allowed researchers to achieve picosecond and subpicosecond pulses.
Researchers in the 1960s and 1970s were flabbergasted with their inability to produce pulse peak powers of intensities exceeding gigawatts/cm2, given that such high-powered pulses caused serious damages to the gain medium. Strickland and Mourou recognized the potential of CPA—first applied to radars in the 1960s to increase their power—to vastly increase laser pulse power, and so they brought CPA to lasers in the 1980s. In CPA, an ultrashort laser pulse is stretched out in time prior to introducing it to the gain medium using a pair of gratings. The low-frequency component travels a shorter path than does the high-frequency component, such that the pulse is “stretched” and its intensity is reduced. The pulse is then safely introduced through the gain medium, amplified, and then recompressed back to the original pulse width, achieving orders of magnitude higher peak power than lasers could generate prior to CPA, without deleterious effects to the laser itself. 4
Moulton’s demonstration of laser quality titanium-doped sapphire crystals in the mid-1980s represented another timely advance in FSL development. Within a few years, Sibbett and associates used the Ti:sapphire system and a concept dubbed Kerr-lens mode-locking to produce sub-hundred femtosecond pulses. 5 Through this process, short optical pulses propagate through a nonlinear medium, such as Ti:sapphire, resulting in a significantly large number of modes oscillating in synchrony with each other to generate pulses in the femtosecond regime, similar to what we described previously. The FSL was thus born.
2.4 Femtosecond Laser in Medicine
The FSL has multiple applications within medicine, including, but not limited to, imaging and nanosurgical manipulation on the cellular and subcellular level, 6 neurosurgical and cardiovascular interventions, 7, 8, 9and dental procedures. 10 On a molecular level, femtosecond pulses have been used to elucidate the mechanisms of chromosome separation during cell division, to induce highly localized DNA damage, to measure the biophysical properties of the cytoskeleton and mitochondria, and to stimulate calcium waves in living cells. 6
Importantly though, the FSL may someday extend itself to the treatment of neurological diseases. In one study, researchers used the FSL to perform very precise axotomy in Caenorhabditis elegans to study nerve regeneration. 11 Researchers cut axons to impair the worms’ backward motion and showed that these axons regenerated functionality within 24 hours of the operation, as the worms recovered backward movement. Furthermore, researchers at Cornell injected 4-aminopyridine (a seizure-inducing drug) locally into cortical rat tissue and stopped focal seizure propagation with the use of precise and controlled FSL incisions without disrupting normal neural functionality. 7
The FSL may also benefit patients with cardiovascular disease, given its promise to reduce restenosis in drug-eluting stents and to allow for the fabrication of microvessel scaffolds. 8, 9 Researchers have used laser-assisted patterning to modify the stent surface, allowing them to control cell–surface interactions that play a major role in the restenosis. They observed that myofibroblast proliferation decreased significantly on laser-treated samples in comparison to nontreated ones, suggesting that the FSL may be used to modify stent surfaces for prevention or at least reduction of restenosis. 9 The applications of the FSL in medicine are shown in ▶ Table 2.1.
Field | Use |
Molecular and cellular biology |
|
Neurology |
|
Cardiology |
|
Dentistry |
|
2.5 Introduction of the Femtosecond Laser in Ophthalmology
The FSL represents a major paradigm shift. It was first introduced to ophthalmic practice in 2001 as a means of creating a corneal flap during laser-assisted in situ keratomileusis (LASIK). Since then, it has found other uses across multiple ophthalmic domains. The FSL applies a highly focused, pulsatile laser beam to a target tissue, generating plasma consisting of free electrons and ionized molecules that rapidly expand and collapse, resulting in microcavitations and acoustic shock waves that separate and incise target tissue. The FSL employs ultrafast pulse times of 10–15 s, allowing for smaller amounts of energy to be used while maintaining similar power output, thus sparing delicate, adjacent tissues from collateral damage. Furthermore, light of 1,053-nm wavelength is not absorbed by optically clear tissues at low power densities and is unaffected by corneal magnification, permitting precise focusing of a 3-μm spot within the anterior chamber, accurate to within 5 μm. To a limited degree, the FSL is also capable of passing through optically hazy media such as edematous cornea and even the relatively translucent perilimbal sclera. 1
A little shy of a decade passed until the FSL translated from early experimental investigation to meaningful ophthalmic application. Realizing that the clinically available nanosecond laser systems in the 1990s required large pulse energies to achieve optical breakdown, leading to uncontrolled and undesirable tissue effects, Tibor Juhasz and Ron Kurtz at the University of Michigan decided to explore how lasers capable of generating even shorter laser pulses would benefit the performance of minimally invasive and highly localized corneal procedures. An endowment from the National Science Foundation and the state of Michigan enabled them to construct a prototypical, solid-state Nd:glass laser system that produced 500-fs pulses at a wavelength of 1.06 μm. They demonstrated flap creation, keratomileusis, and intrastromal vision correction with their prototype and noted unparalleled precision and lack of collateral tissue damage, which had previously been unattainable with nanosecond lasers. 12, 13
Juhasz and Kurtz immediately recognized their potential to effect a paradigm shift within ophthalmology. They went on to found IntraLase Corp with the intention of improving LASIK and other refractive procedures. They approached William Link, who had previously founded American Medical Optics and Chiron Vision. Link convinced the two to move to Irvine, CA, a place he felt would be more nurturing to start-up companies such as IntraLase. The Food and Drug Administration (FDA) approved IntraLase for lamellar corneal surgery in 2000, and then it became commercially available in 2001 for LASIK flaps. While the system initially operated at a frequency of 6 kHz and then 10 kHz, IntraLase quickly improved its FSL to reduce procedural times and to improve outcomes as well as ease of use. They introduced a 15-kHz model in 2003, followed by the 30-kHz model in 2005 and the 60-kHz model in 2006. Flap creation time with the 60-kHz model rivaled that of the mechanical microkeratome and was even further improved with the new 150-kHz fifth-generation IntraLase, which creates a flap in less than 10 seconds and can cut a wide variety of geometric shapes, depths, diameters, wound configurations, spot sizes, and spot separation. Advanced Medical Optics (AMO) bought IntraLase in 2007, which Abbott Labs later acquired in 2009. 13, 14
Kurtz left IntraLase before the company was sold to AMO and founded LenSx Lasers in 2008, with the intention of enhancing cataract surgery with the FSL. Backed by venture capitalists, Kurtz built the LenSx system, which operates at a frequency of 50 kHz and has combined 3D spectral-domain optical coherence tomography (OCT) to enable image-guided cataract surgery. Stephen Slade performed the first laser cataract surgery on 50 consecutive eyes with LenSx in 2010 and noted essentially perfect anterior capsulotomy centration. LenSx received FDA approval in the United States as well as CE (European Conformity) approval in Europe for corneal and arcuate incisions, anterior capsulotomies, lens fragmentation, and corneal flap formation. In a move to bolster its pipeline and stake its ground in the coveted FSL-cataract surgery market, Alcon entered into an agreement to acquire LenSx. 13, 14
Entrepreneur Randy Frey founded Lasersoft Vision in 2004 and later teamed up with venture capitalist Aisling Capital. Together, they developed an 80-kHz FSL called LensAR, initially to treat presbyopia by using femtosecond pulses to restore the power and flexibility of the natural crystalline lens. However, Frey’s medical advisors observed that the laser was better suited to removing lenticular material, which they felt could be useful during complicated high-grade cataract cases. Thus, the company changed its name to LensAR in 2007 and refocused its efforts on cataract surgery. Initial clinical results showed that the anterior capsulotomies created by LensAR were regular and well centered, that patients’ vision rapidly recovered after surgery, and that less ultrasound energy was necessary while phacoemulsifying high-grade cataracts. By May 2010, the FDA cleared LensAR to create anterior capsulotomies and, less than a year later, to perform lens fragmentation. LensAR also has FDA and CE approvals for corneal and arcuate incisions. 13, 14
The founders of one other company, OptiMedica, felt more intrigued by the possible extensions of the FSL to retina and glaucoma surgeries and so they developed Catalys, which was a behind-the-scenes effort. After investors saw the potential of FSL anterior capsulotomy, however, OptiMedica sold its retina and glaucoma assets in 2010 and began to focus on cataract surgeries. Operating at 120 kHz, Catalys has a combined 3D spectral-domain OCT enabling image-guided cataract surgery, and it currently has FDA and CE approval for corneal and arcuate incisions, anterior capsulotomies, and lens fragmentation. 13, 14
A more recently unveiled FSL, VICTUS, introduced by Tecnolas in 2011 at the European Society of Cataract and Refractive Surgery (ESCRS) meeting, is the first FSL capable of performing both cataract and refractive surgery on a single platform, operating at 80 kHz during cataract procedures and 160 kHz during refractive surgeries. VICTUS features 3D spectral-domain OCT to enhance surgical planning and monitoring, and currently has FDA and CE approvals for corneal and arcuate incisions, anterior capsulotomies, lens fragmentation, and corneal flap creation. Tecnolas has entered into a co-promotion agreement with Bausch and Lomb in 2011, which includes an option for Bausch and Lomb to purchase the company should the laser meet certain key milestones. Since that time, both companies have leveraged their combined cataract and refractive surgery experience to promote VICTUS. 13, 14
Other FSL systems within the U.S. market include Tecnolas FEMTEC, Ziemer Femto LDV, and Zeiss VisuMax. The FEMTEC operates at either 40 or 80 kHz and has FDA approval for corneal flap creation and for use in cataract surgery. A recent 36-month follow-up study involving 20 eyes receiving intrastromal ring cuts demonstrated improvement in uncorrected near vision, suggesting FEMTEC is useful in presbyopia treatment. 15 Femto LDV, developed by Ziemer in 2005 and marketed originally as DaVinci until its trademark became a concern, holds the distinction of being the fastest FSL approved in the United States with a pulse rate in the megahertz range. This FSL has been approved for LASIK flap creation and has been used to create pockets for presbyopic inlays, to create circular tunnel incisions for intrastromal ring segments, and to perform lamellar keratoplasty. Another FSL, the Zeiss VisuMax, has CE approval for femtosecond lenticule extraction (FLEx) in Europe. 16 Both FLEx and small-incision lenticule extraction (SMILE) are refractive procedures involving intrastromal ablation, and provided that extensive investigation proves their safety, efficacy, and long-term stability, they could become direct competitors to LASIK. The applications of the FSL in ophthalmology are shown in ▶ Table 2.2.
Field | Use |
Corneal and refractive surgery |
|
Cataract surgery |
|
Intraocular lens technology |
|
Glaucoma surgery |
|
Retinal surgery |
|
Abbreviations: DLEK, deep lamellar endothelial keratoplasty; DSAEK, Descemet’s stripping automated endothelial keratoplasty; FLEx, femtosecond lenticule extraction; FSL, femtosecond laser; LASIK, laser-assisted in-situ keratomileusis; MIGS, microinvasive glaucoma surgery; SMILE, small-incision lenticule extraction. |
2.5.1 Femtosecond Laser in Corneal and Refractive Surgery
The FSL is most commonly used to create LASIK flaps. During this procedure, the surgeon docks the patient’s eye to a low-pressure suction ring to align the globe and flatten the cornea, after which she or he fires FSL pulses at the cornea stroma at a predetermined depth and in a raster or spiral pattern to create the lamellar cut and then in a peripheral circular pattern to create the vertical side cuts. Compared to microkeratome-based LASIK, FSL-based LASIK reduces the incidence of flap complications; gives surgeons more options regarding flap diameter and thickness; improves precision, flap safety, and thickness predictability; and does not have moving parts. Adjusting FSL parameters allows surgeons to perform anterior lamellar keratoplasty and to produce penetrating keratoplasty cuts with shaped graft–host junctions, posterior donor lamellar buttons in deep lamellar endothelial keratoplasty (DLEK) and Descemet’s stripping automated endothelial keratoplasty (DSAEK), tunnels for intracorneal ring insertion (Intacs), flaps for corneal lenticular inlays, arcuate astigmatic keratotomy incisions, and cuts in recipient corneas for permanent keratoprosthesis implantation. 17, 18
Animal and human studies on FSL-assisted anterior lamellar keratoplasty show promise, as do eye bank and animal model investigations on FSL-assisted posterior lamellar dissection techniques. Initial histologic studies on posterior lamellar techniques demonstrated the laser’s ability to produce smooth lamellar cuts with straight trephination edges; but subsequent scanning electron microscopy studies revealed irregularities of the lamellar surface texture thought to be due to laser scatter and attenuation in the deep stroma. Additional ongoing eye bank investigations include FSL-cutting of donor posterior corneal buttons for DSAEK; FSL-extraction of donor corneal tissue and application of donor tissue to recipient cornea during penetrating keratoplasty; and FSL-microkeratome creation of cornea pockets to facilitate the insertion of biopolymer keratoprostheses in eye bank corneas. As these procedures are undergoing preliminary investigation, associated safety issues and complications are unknown as of yet. Another intriguing application of the FSL, according to one report, is that it has been used to obtain diagnostic corneal biopsies in cases of suspected infectious keratitis. 17, 18
The FSL may lend itself well to treating keratoconus and myopia (up to -3.5 D) by facilitating intrastromal implantation of polymethylmethacrylate (PMMA) implants, and to treating astigmatism after penetrating keratoplasty (PKP) or cataract surgery. FSL-tunnel creation for intrastromal PMMA implants is reportedly easier, more precise and predictable, less likely to perforate the cornea, and results in improved visual outcomes, when compared to the conventional mechanical spreader. Furthermore, correction of high astigmatism following PKP or cataract surgery via laser-assisted arcuate keratotomy and/or wedge resection is easier, more precise, and carries less risk of corneal perforation than the free-hand diamond blade method. 17, 18
New intrastromal ablation procedures made possible by the FSL (FLEx and SMILE) may revolutionize refractive surgery and render LASIK obsolete. Sekundo developed FLEx in 2008 following the introduction of the VisuMax FSL in 2007. This procedure involves a single FSL—as opposed to two lasers in LASIK—that cuts a precise lenticule of tissue completely contained within corneal stroma, as well as a corneal flap for lenticule extraction. A 5-year follow-up study indicates that FLEx provides safe and stable long-term refractive benefits for myopic and astigmatic patients. A newer, more novel technique (SMILE) also involves cutting an intrastromal lenticule, but this procedure does not require a corneal flap for extraction. Given that the lenticule is extracted through a peripheral incision, SMILE is less invasive and, when compared to LASIK, promises to improve corneal biomechanical stability and to avoid the postoperative complications associated with flaps. According to one review, SMILE has produced an efficacy, predictability, and safety profile similar to that of LASIK, yet advantages related to improvement in biomechanical stability, postoperative inflammation, and dry eye have not been fully established. 19 FLEx has been approved in Europe, but both FLEx and SMILE are pending FDA approval.
2.5.2 Femtosecond Laser in Cataract Surgery
Just as it has done for LASIK and other refractive procedures, the FSL can deliver the necessary accuracy and precision during cataract surgery to improve beyond current clinical outcomes. To date, FSL systems are engineered to perform clear corneal incisions (CCIs), capsulotomies, lens fragmentation, and limbal relaxing incisions (LRIs) (▶ Fig. 2.1 and ▶ Fig. 2.2).
(Reproduced with permission from Mamalis N, Werner L, Farukhi MA, Kramer GD, MacLean K. Fun with femtosecond lasers. Video presented at the American Society of Cataract and Refractive Surgery (ASCRS), San Diego, CA, April 2015.)
Fig. 2.1 Eye prepared according to the Miyake–Apple technique (posterior view of the anterior segment) and submitted to cataract surgery with a femtosecond laser. (a) Anterior capsulotomy. (b) Nucleus fragmentation with a grid pattern. (c) Corneal incision (arrow).
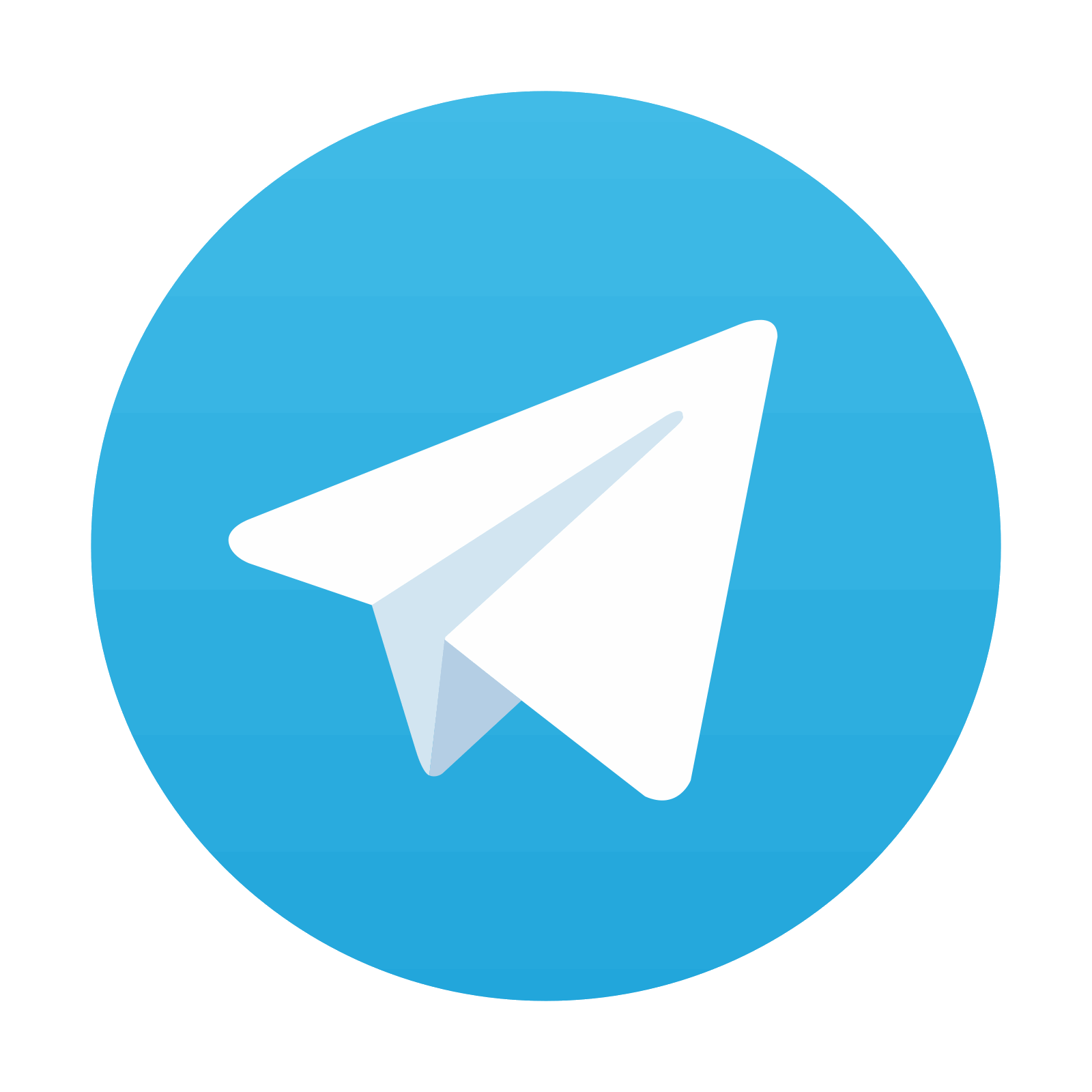
Stay updated, free articles. Join our Telegram channel
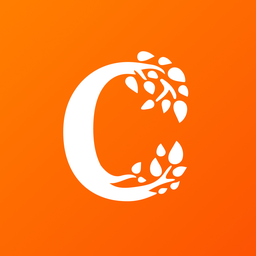
Full access? Get Clinical Tree
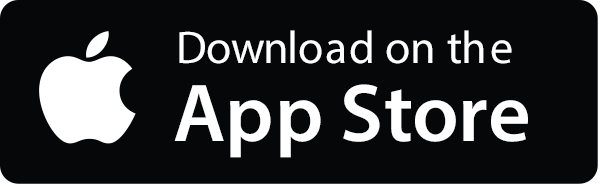
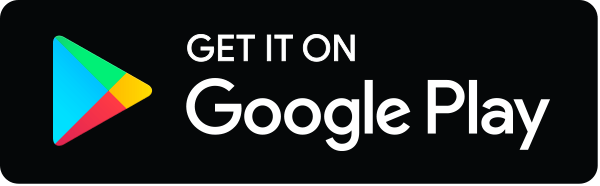