© Springer International Publishing Switzerland 2017
Jorge L. Alió (ed.)KeratoconusEssentials in Ophthalmology10.1007/978-3-319-43881-8_1212. Role of Corneal Biomechanics in the Diagnosis and Management of Keratoconus
(1)
Eye Hospital, WenZhou Medical University, Wenzhou, 325027, China
(2)
The Institution of Ocular Biomechanics, Wenzhou Medical University, Wenzhou, Zhejiang, 325027, China
(3)
School of Engineering, University of Liverpool, Liverpool, L69 3GH, UK
(4)
NIHR Biomedical Research Centre for Ophthalmology, Moorfields Eye Hospital NHS Foundation Trust and UCL Institute of Ophthalmology, Liverpool, UK
Keywords
KeratoconusIn vivoCorneal biomechanicsDiagnosisManagementCorneal collagen cross-linking12.1 Introduction
Keratoconus (KC) , the most prevalent form of idiopathic corneal ectasia, is a progressive degenerative eye disease characterised by localised thinning and conical protrusion of the cornea. The cone typically develops in the inferior-temporal and central zones [1] although superior localisations can also occur [2]. Consequently, visual acuity is reduced due to irregular astigmatism and high myopia resulting from the corneal topographical changes. KC affects both genders and all ethnicities [3–6]; however, higher incidence has been reported in Asians when compared to Caucasians [7, 8]. Onset usually occurs during puberty and progresses until the fourth decade of life [3]. With 50 % of unilateral cases progressing to bilateral KC within 16 years [9], experts now agree that true unilateral KC does not exist [10].
Although the aetiology and pathology of the disease is still not fully understood, various biochemical, cellular and microstructural differences have been reported in the literature. For instance, biochemical changes include increased activity of proteolytic enzymes and a decrease in their inhibitors [11, 12]. A progressive reduction in collagen producing keratocytes has also been observed [13] and it has been suggested that variations in collagen type XIII [14], XV and XVIII [15] may alter the healing properties of keratoconic corneas. Stromal ultrastructural abnormalities comprise altered spatial distribution of proteoglycans [16], changes in collagen organisation and uneven distribution of collagen mass [17] as well as decreased fibril diameter and interfibrillar spacing, undulation of collagen lamellae [18], reduced lamellar interweaving and loss of lamellae inserting into Bowman’s layer [19]. Moreover, the observed thinning of the stroma in KC has been associated with the uneven distribution of collagen mass with inter- and possibly intra-lamellar displacement and slippage resulting in changes in corneal curvature [20, 21].
12.2 Clinical Tools for the Study of Corneal Biomechanics in Keratoconus: Diagnostic Techniques
With the disruption of the collagen network, the intraocular pressure causes a weakened cornea to bulge from its normal shape and become progressively conical. Consequently, corneal topography has become the most widely used tool to detect KC. Abnormal corneal tomography parameters such as atypical pachymetry profile, irregular anterior curvature and increased posterior surface elevation have all been used to detect KC at different stages of the disease. While topography analysis is well suited to characterising KC when clear geometrical changes have occurred in the cornea, its robustness reduces when attempting to assess mild, pathologic cases, especially in subclinical or early KC [22]. The reduced efficacy of these techniques stems from the fact that changes in corneal geometric features are secondary signs of KC whereas the earliest initiating changes of the disease occur within the microstructure and hence the biomechanical properties of tissue. Since KC is thought to be associated with a “weaker” cornea, a technique capable of determining in vivo biomechanical behaviour of this ocular component may be an important tool which could be used in the detection of subclinical KC [23].
12.2.1 Corneal Biomechanical Properties
The cornea exhibits complex biomechanical behaviour characteristics, namely hyperelasticity (a nonlinear stress increase with a linear strain increase), viscoelasticity (hysteresis, creep and stress relaxation) and anisotropy (directionally dependent response to applied loads). Non-enzymatic glycosylation, or “cross-linking”, of collagen molecules also results in age-dependent stiffening of the tissue. Furthermore, medical history and diseases such as KC as well as treatment methods can also affect the biomechanical characteristics of the tissue. As a result, accurately determining the biomechanical behaviour of the cornea requires significant effort and its difficulty is exasperated when in vivo characterisation is considered. Nevertheless, there has been significant scientific interest in assessing corneal biomechanical properties due to potential clinical applications in recent years, particularly in the diagnosis and management of KC.
Since biomechanical stability is dependent on the regulation and organisation of structural components within the cornea, the biochemical, cellular and microstructural changes observed in keratoconic corneas would be expected to have negative consequences on structural integrity of the tissue and alter its biomechanical properties [24]. Tensile properties of soft biological tissues are determined by the size and organisation of collagen fibrils. Therefore, the observed alterations in alignment, diameter and spatial order of fibrils in KC would undoubtedly have implications on the cornea’s response to intraocular pressure (IOP) induced stress. The corneal epithelium undergoes the earliest pathological changes in KC and proteolytic enzymes released by degenerating basal epithelial cells cause instability of Bowman’s layer and loss of stromal collagen fibrils. In cases of acute corneal hydrops in KC, abnormalities in Descemet’s membrane and endothelium have been noted. All of these combined may be responsible for the biomechanical instability of the tissue in keratoconus.
Experimental studies of ex vivo KC corneas have reported abnormalities in biomechanical response to applied loads when compared to normal corneas [25, 26]. However, in vivo measurement of corneal biomechanics still remains a difficult task. There are currently only two commercially available instruments capable of quantifying in vivo biomechanical metrics that can be used to assist in the diagnosis of the KC. These instruments are summarised as follows.
12.2.2 Ocular Response Analyser
The Ocular Response Analyser (ORA) became commercially available in 2005 and was the first device capable of evaluating the biomechanical response of the cornea in vivo. The device quantifies the dynamics of corneal deformation and recovery during a variable air-puff pressure application over a 20 ms period. In addition to intraocular pressure and pachymetry readings, the device also provides two biomechanical metrics: corneal hysteresis (CH ) and corneal resistance factor (CRF ). CH is the difference between the two applanation pressures (P 1 and P 2) recorded while applying the air puff. CRF, on the other hand, is an indicator of the overall resistance of the cornea to the applied air-puff pressure and is significantly correlated with central corneal thickness (CCT). Both of these biomechanical metrics are influenced by the viscoelastic behaviour of the corneal tissue [27]. Clinically measured metrics provided by ORA have been widely used to assess the biomechanical response of the cornea in order to help identify potential cases of KC. Compared with normal patients, both CH and CRF are usually reduced in KC corneas indicating a mechanical weakening of the stroma [28]. However, a wide substantial overlap exists in the biomechanical metrics of normal and keratoconic corneas [29, 30] and so they have not been as effective in identifying KC as first anticipated. Furthermore, the exact correlation between these metrics and the established mechanical properties of tissue (such as tangent modulus) is still unknown [22]. Thus, the ORA must be complemented with other diagnostic imaging tools to obtain a reliable diagnosis of KC. With the introduction of a software update (version 2.0) in 2009, the ORA now computes 37 new parameters that describe the waveform of the ORA applanation signal. These parameters show promise in providing additional biomechanical information about KC corneas [31, 32]. However, the manufacturers have not yet provided a specific explanation of the meaning of each parameter and so they still require thorough clinical validation before they can be commonly used in clinical practise.
12.2.3 Corvis ST
The Corvis ST (CVS ) is another representative non-contact device that was first introduced in 2010. The CVS employs a similar deformation technique to the ORA, but with a non-varying maximum air pressure and provides information about the biomechanical response of the cornea using dynamic Scheimpflug imaging analysis. The CVS captures approximately 140 cross-sectional images of the cornea during the air-puff-induced dynamic deformation [33] using its high-speed camera system. This information is then used to characterise the morphological response of the cornea to the instrument’s air-puff pressure using ten deformation parameters, some of which are strongly correlated with the tissue’s mechanical stiffness. As shown in a previous study, the maximum deformation amplitude of keratoconic corneas is much greater than that of normal corneas [34]. Further analysis of the CVS data may yet be used to yield additional metrics about the biomechanical status of the cornea. However, the usefulness of CVS to evaluate KC severity and diagnose subclinical KC is yet to be determined. The inclusion of a high-speed Scheimpflug camera allows for precise monitoring of in vivo cornea cross-sectional deformation under the applied air pressure. This deformation data provides biomedical engineers with invaluable information that can be used to determine more precise biomechanical properties of the tissue. Work is now progressing to utilise this device to produce regional estimations of in vivo corneal stiffness, which may allow for better planning of the treatment and management of KC .
12.2.4 Other Devices
Several other technologies have also been developed to evaluate corneal biomechanical parameters in vivo such as optical coherence tomography [35], supersonic shear wave imaging (SSI ) [36], confocal microscopy [37], applanation resonance tonometer (ART ) [38], acoustic radiation force (ARF ) [39] and scanning acoustic microscopy [40]. However, the validation of these technologies in human eyes will be essential before using the findings of studies to help improve the accuracy of KC diagnosis. Lack of reliable in vivo measurements or devices capable of characterising true corneal material properties has meant that KC biomechanics have only been investigated to a limited extent. It is now becoming apparent that the bulk biomechanical assessment of the cornea may not be sufficient to fully characterise this typically asymmetric disease. Spatial location of focal weakening in the cornea may be necessary to detect the disease at its earliest stages as well as fully characterise its progression.
12.3 Keratoconus Treatment Techniques: Implications of Corneal Biomechanics
KC is currently managed using a number of methods ranging from non-invasive options capable of providing short-term results to invasive techniques for more long-term outcomes. However, the method of treatment used is highly dependent on the severity of the ectasia. In early stages for instance, spectacles can sufficiently correct refractive errors although this method becomes unsuitable for correcting the irregular astigmatism associated with KC as the disease progresses [3]. In mild to moderate KC, contact lenses, especially rigid gas permeable (RGP) lenses, are the most common and successful method of treatment providing improved visual acuity whilst decreasing the need for surgical interventions [41]. In contrast to the aforementioned short-term solutions, which aim to improve visual acuity by improving the anterior curvature of the cornea, more long-term invasive clinical interventions are also available. Intrastromal corneal ring segment (ICRS) implants and corneal cross-linking (CXL) aim to improve the shape of the cornea or halt the progression of the cone. For advanced cases, which cannot be successfully managed with regular treatment, deep anterior lamellar keratoplasty (DALK ) and penetrating keratoplasty (PK) were introduced to replace either the anterior layers of the stroma or the entire cornea with healthy donor tissue, respectively.
12.3.1 Contact Lenses
Contact lenses aim to improve the anterior curvature of the cornea and increase visual acuity. Nevertheless, all options interact mechanically with the cornea to varying degrees. While several lens options are available, the most commonly used materials include soft hydrogel and silicone hydrogel , and RGP polymethylmethacrylate (PMMA). Although soft lenses provide increased comfort for the wearer, rigid lenses are more prevalent since high levels of irregular astigmatism cannot be corrected with other lens types [42, 43].
Soft lenses interact mechanically with the cornea as surface tension forces arising from the tear film at the lens periphery and a negative pressure within the tear reservoir between the lens and cornea attract the lens towards the eye [44, 45]. Bespoke lens options, such as the KeraSoft® lens , are individually lathe cut to fit the specific irregularity of a patient’s cornea resulting in a close fit between the lens and eye while improving the anterior curvature. The Young’s modulus of current silicone hydrogel lenses is typically in the region of 0.3–1.0 MPa [46] which is similar to the tangent modulus of the cornea under normal IOP [47]. Consequently, the effect of the forces acting between the contact lens and eye influences the final lens topography and hence affect the patient’s visual acuity. However, this mechanical interaction and subsequent change in lens topography is not yet considered when designing soft lenses.
As with soft lenses , RGP lenses are held in place by forces generated between the tear film, lens and eye but the degree of fit between the lens and eye varies. In mild KC an ideal fit can be achieved and the lens is usually intended to rest on the apex of the cone. However, as the cone progresses a compromised fit may need to be accepted as long as it does not cause damage to the cornea. In this instance, additional mechanical action occurs between the lens and cornea as the lens presses against the cone and temporarily changes its shape [48]. The Young’s modulus of the PMMA material used in RGP lenses is also three orders of magnitude higher than that of soft lenses. Consequently, mechanical interaction with the cornea would be unlikely to cause any significant changes in lens shape. Originally it was hoped that the lens bearing pressure on the cornea could correct or stabilise the ectasia by flattening the cone [49]. However, it was later found that this can result in abrasion and scarring of the cornea [50].
12.3.2 Intrastromal Corneal Ring Segments
ICRS implants are designed to reshape abnormal corneal topographies based on an ‘arc-shortening effect’ when introduced into the stroma. This method was first developed to correct low myopia during its early stages [51] but has now become a treatment option for KC patients with significant irregular astigmatism and an intolerance to RGP lenses [52]. It has been shown that eyes with varying severities of KC respond differently to ICRS placement with the greatest effect shown in mild to moderate KC [53]. ICRS implants are inserted into the stroma by creating an incision using a femtosecond laser or a mechanical tunnelling technique in the peripheral region of the cornea with the aim of decreasing asymmetrical astigmatism and convexity of the cone [54, 55]. However, the incisions required to insert the implants would be expected to lead to a relaxation of the anterior stromal tissue resulting in an altered shape. Furthermore, the effect of wound healing may lead to a possible increase in membrane stiffness of the cornea whereas tissue separation caused by the introduction of the implant could result in changes in flexural stiffness. Although there have been no statistically significant differences observed in cornea hysteresis (CH) and corneal resistance factor (CRF) parameters obtained from the ORA [56], the introduction of rigid components to the stroma would be expected to affect the biomechanical behaviour of the tissue. This could be due to tissue scarring within the stroma resulting from the introduction of the ICRS implants or changes in the overall mechanical response of the tissue particularly in the peripheral region where the implant has been introduced.
12.3.3 Cornea Collagen Cross-Linking
CXL is achieved via saturation of the stroma with riboflavin followed by irradiation of the central region of the cornea using ultraviolet-A light at 365–370 nm [57]. While stromal saturation originally required the removal of the corneal epithelium, more recent advances in techniques allow for transepithelial application of riboflavin. Nevertheless, in all cases UVA is applied centrally with uniform distribution and in the same form for all patients despite their disease state. The procedure activates riboflavin generating reactive oxygen species that induce cross-links at the surface of collagen fibrils and within the proteoglycan-rich coating surrounding them [58] as well as limited linkages among collagen molecules and among proteoglycan core proteins [59]. The outcome of this procedure is an overall increase in mechanical stiffness [60] which usually halts the progression of the cone [61]. While various options are now available for the management of KC, CXL is fast becoming the most commonly used technique. This technique can be used to halt progression of the cone at all stages of the disease [10] provided that the minimum stromal thickness is at least 400 μm, thereby reducing further degradation of visual acuity and limiting the need for corneal transplants. With the development of CXL , some patients who might otherwise have required penetrating keratoplasty are now able to undergo a relatively well-tolerated procedure to potentially stabilise the progression of their disease. However, the uniform application of the CXL treatment to the diseased cornea will result in an over-stiffening of the regions unaffected by KC, something that is not yet considered in the patient treatment.
12.3.4 Keratoplasty
Advanced cases of keratoconus, such as keratometry values steeper than 55 D, corneal astigmatism >10 D, corrected visual acuity worse than 6/12 (20/40), the presence of corneal scarring or poor contact lens tolerance may require PK [62–64]. The procedure entails removing the entire thickness of the cornea, which is then replaced with donor tissue [3, 62]. DALK , in which anterior corneal layers are removed and replaced with healthy donor tissue while Descemet’s membrane and the endothelium remain intact [65, 66], was employed in KC management in recent years. Compared with PK, DALK has a lower risk of endothelial cell loss and graft rejection [65], avoids the risk associated with open sky surgery such as expulsive haemorrhage, endophthalmitis, iris and/or lens damage, and offers superior wound strength [67, 68]. For corneal pathologies not affecting the endothelium and Descemet’s membrane, DALK is a reasonable alternative to PK [67]. However, normal stromal architecture cannot be fully recovered in full-thickness graft wounds [69–71]. Abnormalities in collagen fibril orientation and spatial organisation have been found around the entire graft margin following PK which may affect corneal biomechanical behaviour and graft stability in the long term [72].
12.4 Biomechanical Changes Induced by the Use of the Different Therapeutic Modalities
Although all current KC management techniques involve mechanical interaction with or mechanical changes to the cornea, the design and planning of these interventions do not consider the mechanical properties of the cornea either pre- or post-intervention. For instance, hypoxia-related corneal oedema can occur as a result of prolonged soft lens wear [73] even though the materials used have high oxygen permeability. From a biomechanical perspective, this oedema can lead to a decrease in corneal stiffness and hence an increase in corneal deformation [74]. In rigid lens wear, where the interaction between the lens and cornea can be even more pronounced, changes in corneal shape with possible biochemical, cellular and microstructural responses may have subsequent consequences for the overall biomechanical integrity of the cornea.
Surgical interventions can also result in biomechanical changes to the cornea. Recent studies using the ORA have found that CH and CRF values from DALK-treated corneas are similar to normal corneas whereas the corresponding values for PK-treated corneas are significantly lower [75, 76]. This reduction in the values of these metrics indicates a softer cornea. It has been suggested that this weakening is the result of lasting changes in collagen fibril orientation in and around the PK wounds caused by incomplete stromal wound remodelling. Conversely, the improved structural integrity of DALK-treated eyes may be the result of combined healing at the deep interface and graft margin as well as the intact Descemet’s membrane.
The most significant iatrogenic changes in KC corneal biomechanics are those observed when a patient has been treated using CXL. Experimental cross-linking studies have reported human corneal stiffness increases in the region of 300 % using riboflavin/UVA treatment [60] but surprisingly no change in interlaminar cohesion [77]. However, in vivo assessments of corneal biomechanical stress–strain behaviour properties have not yet been determined as it is currently not possible to obtain this information. Consequently, this has meant that clinicians have to make assumptions about the post-procedure mechanics of the tissue, which can result in outcomes that are less than perfect. Furthermore, when riboflavin is combined with UVA irradiation, the cytotoxic effect is ten times greater than UVA irradiation alone [78]. It is therefore important to ensure that sufficient increases in tissue stiffness are achieved without damaging cellular components as complications from the procedure may result in the need for keratoplasty .
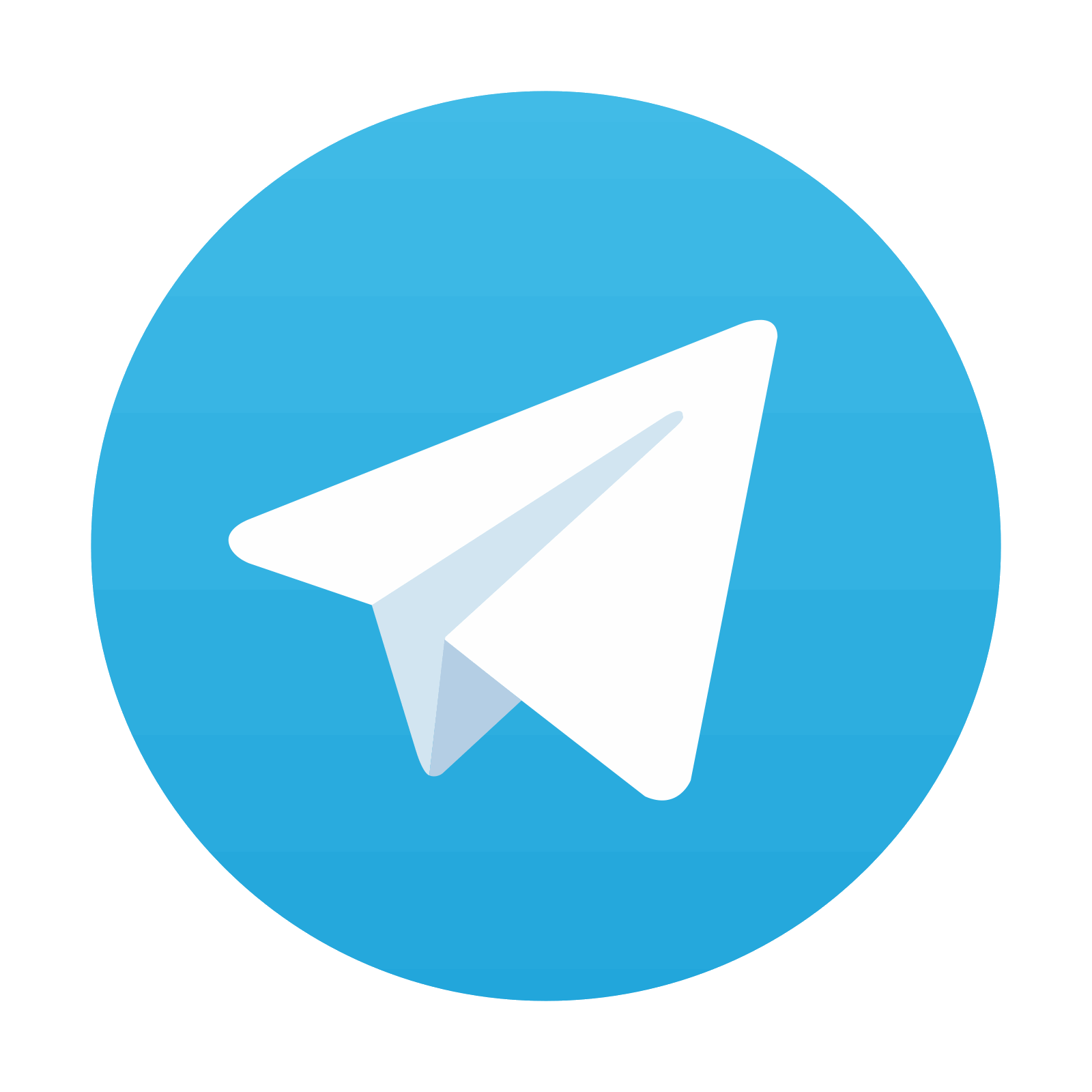
Stay updated, free articles. Join our Telegram channel
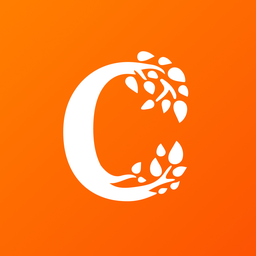
Full access? Get Clinical Tree
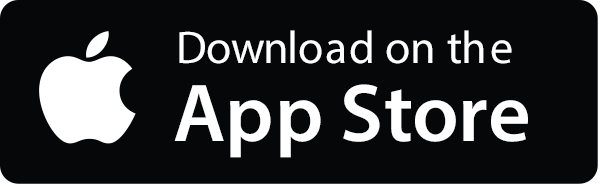
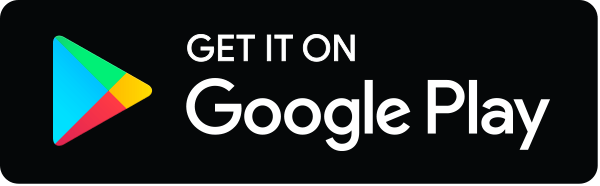