Fig. 1.1
The two-process model of sleep. Green arrows denote the circadian alerting signal; the longer the arrow, the greater the alerting signal. Purple arrows denote the homeostatic sleep drive. The longer the arrow, the greater the homeostatic sleep drive. The blue vertical line divides wake from sleep. The level of alertness is represented by the point at which the arrows meet, determined by the net effect of circadian and homeostatic forces. Propensity for sleep is high when either the magnitude of homeostatic sleep drive overcomes the circadian alerting signal, or the circadian alerting signal is sufficiently low (e.g., at 04:00–06:00). After sleep offset, homeostatic sleep drive accumulates with wakefulness. However, the circadian alerting signal increases in an opposing fashion. However, in the late afternoon the circadian alerting signal is not large enough to completely offset the effect of growing sleep pressure on alertness. Therefore, sleepiness is high at this time, referred to as the afternoon circadian dip. The circadian alerting signal is so high in the evening it overcomes the homeostatic sleep drive. Alertness is high at this time, which is referred to as the forbidden zone for sleep. The circadian alerting signal decreases about 2 h before habitual sleep onset and promotes sleep due to the already elevated homeostatic sleep drive. The initial period of the sleep bout is associated with dissipation of the homeostatic sleep need; however, sleep is maintained due to a persistently low circadian alerting signal, which reaches a nadir around 04:00. Therefore, even when the homeostatic sleep drive is less than the circadian alerting signal, if the circadian alerting signal is low, sleep will continue (as seen during the early morning hours). The above model does not take into account other factors outside of process C and process S mediating alertness
1.2 History of Sleep Recording
Homeostasis, as described by Cannon in 1932, is the need for organisms to achieve a steady state [8]. In 1980, Borbély introduced the term sleep homeostasis, which can be understood by its electrophysiological correlate, slow-wave activity [9]. Descriptions of brain wave activity during sleep began in the late 1930s after the advent of the electroencephalogram (EEG) in 1928 [10]. Recordings demonstrated the following EEG progression after sleep onset: drop out of alpha activity, appearance of low-voltage activity, emergence of high-voltage, low-frequency (delta) waves, followed by an increase in delta waves and the appearance of spindle activity. Thus, delta waves were thought to represent “real sleep” [11, 12]. In addition, delta activity was associated with an increased arousal threshold to auditory stimuli and, therefore, was thought to parallel the depth of sleep [11, 13, 14]. The EEG pattern described above was distinct from the activity recorded in rapid eye movement (REM) sleep described initially by Aserinsky and Kleitman in 1953 [15]. In 1957, Dement and Kleitman introduced a staging schema for EEG activity recorded during sleep. They separated nonrapid eye movement (NREM) from rapid eye movement (REM) sleep, and classified NREM sleep into stages 1, 2, 3, and 4 [16]. Stage 1 sleep was described as low-voltage EEG activity that lacked spindle activity [16]. As sleep continued, the EEG evolved with the development of slower activity, sleep spindles, and K complexes that mark stage 2 sleep [16]. Stage 3 sleep was defined by epochs containing at least two waves greater than 100 uV in amplitude with frequency less than 2 Hz [16]. During stage 4 sleep, the delta waves described above made up more than 50 % of an epoch [16]. Current sleep scoring criteria consolidates stage 3 and 4 sleep into NREM 3 (N3) sleep [17]. In addition to measuring slow-wave sleep as classically defined, the development of EEG spectral analysis allowed for the measurement of slow-wave activity [18]. Slow-wave activity is defined as the EEG power density in the 0.5–4.5 Hz range [18]. Although slow-wave sleep stages and slow-wave activity show considerable overlap, they are distinct constructs and each can generate different insight and opportunity in research and understanding of sleep homeostasis.
1.3 NREM Sleep Anatomy and Physiology
Hyperpolarization of thalamic neurons and synchronization of cortical excitatory and inhibitory postsynaptic potentials are responsible for the high-voltage, low-frequency activity seen on EEG during NREM slow-wave sleep [19, 20]. The sleep-active neurons reside in the ventrolateral preoptic nucleus (VLPO) and median preoptic nucleus (MnPN) of the preoptic area (POA) in the hypothalamus [21, 22]. When active, the VLPO and MnPN inhibit wake-promoting centers via GABAergic and galanergic projections [23–27]. As the wake-promoting centers inhibit the VLPO and MnPN, suppression of their activity releases inhibition of the VLPO and MnPN. Neurons in the VLPO and MnPN demonstrate activity that is most pronounced during NREM and REM sleep, and almost nonexistent during wake [28, 29]. Rates of neuronal discharge from the VLPO parallel the depth of NREM sleep as measured by EEG slow-wave activity [28]. Therefore, this group of neurons may play a significant role in the generation of slow-wave sleep. The inability for both the arousal and sleep-promoting centers to be activated simultaneously underlies the “sleep-switch” hypothesis [30]. This theory helps explain the stability of sleep and wake states such that under normal conditions sleep does not intrude into wakefulness and vice versa [30]. The VLPO and MnPN are regulated by direct and indirect connections from the master clock housed in the suprachiasmatic nucleus (SCN), which facilitates the circadian control of sleep (discussed further in Chap. 9). Figure 1.2 depicts the nuclei responsible for NREM sleep.


Fig. 1.2
Nuclei responsible for the generation of NREM sleep. The orange ovals denote nuclei that are wake promoting when active. Blue rectangles represent nuclei that promote sleep when active. The purple circle is the suprachiasmatic nucleus (SCN) of the hypothalamus, which times sleep and wake. *Projections from the SCN to the VLPO and LH are relayed via the SPZ and DMH (not shown). Green dashed lines illustrate the GABAergic and galanergic pathways from the VLPO/MnPN that inhibit the wake-promoting centers, allowing for sleep. The red dashed lines represent inhibitory efferents to the VLPO/MnPN. With inhibition of the GABA-producing neurons in the VLPO/MnPN by ACh, 5-HT, NE, Gal, and GABA (from the SPZ and DMH), wake-promoting nuclei are disinhibited, which results in alertness. The TMN inhibits the VLPO/MnPN through GABA and Gal opposed to HA, the wake-promoting transmitter of the TMN. VLPO ventrolateral preoptic nucleus, MnPN median preoptic nucleus, LH lateral hypothalamus, TMN tuberomamillary nucleus, DR dorsal raphe, LC locus coeruleus, LDT/PPT lateral dorsal tegmentum/pedunculopontine nucleus, SPZ subparaventricular zone, DMH dorsomedial hypothalamic nucleus, Ach acetylcholine, 5-HT serotonin, NE norepinephrine, Gal galanin, GABA gamma-amino butyric acid [21–29]
As above, the SCN is responsible for timing activity of sleep-generating neurons in the POA such that sleep demonstrates a circadian pattern. However, mechanisms that mediate the homeostatic control of sleep are less clear, but likely involve sleep-promoting neurochemicals (or somnogens) that act directly on the POA [31]. Prostaglandin D2 (PGD2) is a sleep-promoting hormone produced by the leptomeninges and choroid plexus [31]. PGD2 increases during sleep deprivation and acts directly on receptors near the VLPO as well as indirectly by way of other somnogens to induce sleep [31, 32]. Cytokines are also known to elicit sleep and may be most important in increasing NREM sleep during infection [31]. Growth-hormone releasing hormone promotes sleep by its direct action on the POA, and increased secretion of this hormone is seen in association with slow-wave sleep during the initial portion of the sleep period [31]. However, adenosine may be the somnogen most likely to mediate sleep homeostasis as it reflects brain energy levels. Adenosine is produced when adenosine triphosphate (ATP) is broken down in metabolically active tissues [31]. In addition, when cells are fatigued, phosphorylation of adenosine to ATP is reduced [33]; therefore, the greater the neuronal activity, the greater is the rise in adenosine. Animal experiments have demonstrated this with an increase in basal forebrain adenosine levels during wakefulness and a decrease during sleep [34]. Additionally, adenosine increases slow waves on EEG [35]. Adenosine’s effects on sleep are mediated by inhibition of wake-active neurons, disinhibition of sleep-active neurons, and stimulation of sleep-active neurons primarily by action on A1 but also A2a receptors [31]. The alerting effect of caffeine occurs through adenosine receptor antagonism [36].
1.4 Slow-Wave Sleep, Slow-Wave Activity, and Their Dispersion over the Course of a Normal Nocturnal Sleep Period
The early work of Dement and Kleitman demonstrated a cyclical variation of the sleep stages that occurred every 90–100 min [16]. Notably, nearly all high-voltage delta frequency activity took place during the first 3 h of sleep. After the second sleep cycle, stages 3 and 4 sleep were not seen [16]. NREM slow-wave sleep is now well known to decline over the course of subsequent sleep cycles through the night [37]. Apart from slow-wave sleep, slow-wave activity, the density of EEG activity in the 0.5–4.5 Hz range as measured by spectral analysis, demonstrates a similar pattern. Slow-wave activity displays a gradual buildup during the first NREM-REM cycle and an exponential decline from cycle 1 to cycle 3 [18]. Notably, the pattern of slow-wave sleep and slow-wave activity decay described above persists in the nonentrained state, suggesting autonomy from circadian contributions [38, 39].
1.5 Slow-Wave Sleep and Slow-Wave Activity as a Function of Prior Wakefulness
During experiments that evaluated sleep stages during naps taken in the morning, afternoon, and evening, a progressive increase was seen in the amount of slow-wave sleep recorded over the course of the day [40, 41]. This finding does not eliminate the possibility of a circadian effect. However, when healthy volunteers slept during nap opportunities at 10:00, 12:00, 14:00, 16:00, 18:00, 20:00, and 04:00, EEG power density in the delta and theta range increased in proportion to the duration of prior wakefulness [42]. Conversely, latency to sleep onset was shortest at the 16:00 and 04:00 naps, consistent with the known bimodal distribution of sleep propensity [5, 42]. In addition, when nocturnal sleep was recorded after napping, there was a significant reduction of slow-wave sleep and slow-wave activity compared to baseline nocturnal sleep that did not follow a nap [43–45].
Studies that used total sleep deprivation protocols of 40–64 h or sleep restriction to 3 h per night showed a significant increase in the amount of slow-wave sleep recorded during the recovery sleep period. In addition, shorter latency to slow-wave sleep was seen during recovery sleep [14, 19, 37, 46]. EEG spectral analysis demonstrated similar findings for slow-wave activity, with increases in delta and theta EEG power during recovery sleep [19, 39, 47–49]. The increase in lower frequency EEG activity was not confined to stages 3 and 4 sleep but also seen during stage 2 and REM sleep. The enhancement of the delta and theta band activity was maximal during the first NREM-REM cycle and the rate of build-up of slow-wave activity in this cycle was increased [19]. These findings were attenuated during subsequent cycles and on recovery day two [19]. Slow-wave activity during recovery sleep was independent of circadian timing [47]. However, slow-wave sleep did not demonstrate the same autonomy, with a reduction in the expected amounted of slow-wave sleep when the recovery period was placed at 07:00 [47]. The authors postulated that this finding was due to the higher circadian propensity for stage REM sleep at that time, such that REM sleep occurred at the expense of NREM sleep. Therefore, visually scored epochs of slow-wave sleep were not increased as expected, as opposed to slow-wave activity which was elevated in proportion to the degree of sleep deprivation [47]. As prior wakefulness does, selective deprivation of slow-wave sleep without decreasing total sleep time also increases slow-wave sleep and slow-wave activity in later portions of the sleep period and during subsequent recovery sleep periods [42, 50, 51].
In summary, slow-wave activity declines over the course of the sleep period, is dependent on prior wakefulness, rebounds when selectively suppressed, and is independent from the circadian control of alertness. Taken together, these findings strongly suggest that slow-wave activity is a useful EEG marker that reflects sleep homeostasis.
1.6 Topographic Organization of Slow-Wave Activity and Local, Use-Dependent Changes
Slow-wave activity has a topographical organization in the cerebral cortex, with an anterior-posterior gradient such that slow-wave activity is more prominent in frontal than occipital areas [52, 53]. This frontal predilection is most pronounced during the initial NREM sleep period and then declines over subsequent cycles [52, 53]. The anterior-posterior gradient is enhanced during recovery sleep following total sleep and selective slow-wave sleep deprivation [51, 53, 54]. These findings suggest a specific role for frontal brain regions in sleep homeostasis.
In addition to amount of prior wakefulness, the magnitude of activity during wake may affect the homeostatic process occurring during sleep. Physical exercise, novel mental activity, and structured social interactions all increase slow-wave sleep [55–57]. Furthermore, activation during wakefulness of specific brain regions has consequences during sleep. For example, a study by Kattler demonstrated that vibratory stimulation of the right hand during wakefulness increased delta power in the left somatosensory cortex during the first hour of sleep that followed [58]. As sensory stimulation is known to increase cerebral metabolism and blood flow in the corresponding cortical area, this finding suggests that the homeostatic process during sleep may be use dependent [58]. In contrast, among subjects subjected to arm immobilization, slow-wave activity in the contralateral sensorimotor cortex decreased during subsequent sleep [59]. The region-specific decrease in slow-wave activity was proportional to next-day deterioration of motor performance in the same limb [59]. In addition to sensorimotor manipulations, an experiment that used a cognitive task that involved specific cortical areas also resulted in locally enhanced slow-wave activity during the sleep that followed [60]. This local, use-dependent increase in slow-wave activity decayed over the course of the night. When subjects were retested, performance of the task was superior among those whose learning occurred prior to sleep; furthermore, performance correlated with the increase in slow-wave activity in the corresponding brain region [60].
Demonstration of local, use-dependent EEG changes, particularly in the setting of learning, has contributed to a synaptic homeostasis hypothesis, to explain a fundamental purpose of sleep, as advanced by Tononi and Cirelli [61, 62]. This hypothesis is based on the following premises. During wakefulness, neuronal plasticity allows the brain to strengthen and increase synapses as the individual navigates new experiences. However, synaptic potentiation comes at a high cost due to the large amount of physiological resources it requires, and therefore is not sustainable. Synaptic pruning, the selection of which synapses should remain, must occur to conserve energy and allow for future learning. The synaptic homeostasis hypothesis proposes that the purpose of sleep is to mediate this process, and that the footprint of this function within sleep is slow-wave activity [61, 62].
1.7 Sleep Homeostasis Across the Life Span
The homeostatic regulation of sleep may differ with age. Slow-wave sleep and slow-wave activity decline steeply during adolescence, particularly during sleep in the first NREM sleep cycle [63–70]. This pattern indicates that children, in comparison to adolescents, have a more rapid buildup of sleep pressure and need a greater amount of recovery to restore homeostasis [70]. These findings may reflect increased synaptic density in children, and provide further support for the synaptic homeostasis theory [61, 62, 70].
The changes continue throughout the life span, with decreased slow-wave sleep and slow-wave activity in older persons [71]. However, as reviewed comprehensively by Bliwise in 1993, studies that have used sleep deprivation protocols have demonstrated similar recovery sleep in elderly and younger subjects. Interestingly, changes in sleep stages after sleep deprivation are seen only in the first recovery night of older subjects, as opposed to the first and second nights in younger individuals. This suggests that aging does not necessarily impair the homeostatic process regulating sleep loss [71]. In fact, older adults in comparison to younger persons may be less affected by sleep loss, with the caveat that differences in baseline sleep among young and old subjects could in part generate these impressions [72–75]. A recent investigation demonstrated that slow-wave activity not only declines in the elderly, but that this decrement is associated with prefrontal gray matter loss and deterioration in memory consolidation [76]. These findings further support the role of slow-wave sleep and slow-wave activity in cognition.
1.8 Genetic Contributions to Sleep Homeostasis
In addition to age, genetics may affect the homeostatic regulation of sleep and wake. A polymorphism in the adenosine deaminase (ADA) gene (G > A transition at position 22 of the gene’s coding region) is associated with increased amounts of slow-wave sleep and greater slow-wave activity [77–79]. The same polymorphism is associated with increased sleepiness and reduced vigilance in the setting of sleep deprivation [78]. A functional polymorphism in the brain-derived neurotrophic factor (BDNF) gene also affects slow-wave sleep and slow-wave activity in both the baseline and sleep-deprived states [80].
In addition, genes traditionally thought to modify circadian aspects of sleep are now known to participate in homeostatic mechanisms. Animal models with deletions or mutations of the circadian genes Bmal1, Cyc, Clock, Npas2, and Cry show impaired sleep homeostasis [81–85]. Additionally, the clock genes Per1 and Per2 have increased expression in animals undergoing sleep loss [83, 86]. In humans, Per3 polymorphisms are involved in sleep homeostasis [87]. Subjects who are homozygous for the five repeat variable number tandem repeat (VNTR) polymorphism (Per3 5/5), in comparison to subjects with the four repeat VNTR polymorphism (Per3 4/4), show shorter sleep latencies, higher initial amounts of slow-wave activity, and more rapid slow-wave decline over the course of the night [87]. Additionally, when sleep deprived for 40 h, Per3 5/5 subjects have increased slow rolling eye movements and greater deterioration in performance measures [87]. Interestingly, a previous study had demonstrated that the longer repeat polymorphism was associated with a morning circadian phenotype, while the shorter repeat polymorphism was more frequent in evening types and those with delayed sleep phase disorder [88].
Another gene that modifies circadian rhythms, hDEC2, has gained considerable attention [89]. A point mutation in this gene was found in a mother and daughter who both display short sleeper syndrome [89]. Individuals with this condition require shorter sleep durations, have faster buildup of slow-wave activity, and thus may function under a higher NREM sleep pressure [49]. Mice genetically modified to contain the same proline to arginine substitution found in this family also demonstrate longer active periods than mice without the mutation [89]. Therefore, hDEC2 is not only implicated in sleep timing but also modifies sleep duration requirements [89]. These findings suggest that the same molecular machinery influences both process C and process S, functions traditionally thought to oscillate autonomously.
1.9 Clinical Manifestations of Sleep Homeostasis
The homeostatic regulation of sleep has important implications in many different clinical settings. Chronic partial sleep loss is ubiquitous, as almost 40 % of American adults report sleep durations of less than 7 h on workday nights [90]. Sleep pressure builds when sleep is chronically restricted and results in reduced daytime alertness and impaired performance [91]. Shift work, as experienced by about 10 % of the US workforce, can exacerbate sleep deprivation. For example, nightshift workers average 6.1 h of sleep per 24-h period [92]. Increased homeostatic sleep pressure amplifies circadian fluctuations in performance [93, 94]. The greatest functional impairment is seen during the circadian night and is more pronounced during chronic partial sleep loss than during acute sleep deprivation [93, 94]. Repercussions can be grave among nightshift workers who undergo recurrent sleep restriction and attempt wakefulness at the circadian nadir of alertness. Naps may provide an important opportunity to mitigate the effects of increased sleep pressure on alertness and performance [95–99].
Impairment of the sleep homeostatic process may play an important role in certain sleep disorders. In idiopathic hypersomnia, excessive daytime sleepiness occurs despite adequate sleep duration and the absence of observable underlying sleep disruption. This disorder lacks the REM intrusion phenomena seen in narcolepsy. In a study that monitored 24-h EEG in 75 patients with idiopathic hypersomnia, slow-wave sleep persisted later in the night than in normal controls [100]. This observation was more prominent among patients who had idiopathic hypersomnia with long sleep time than it was among patients who had idiopathic hypersomnia without long sleep time [100]. In addition, daytime sleep episodes contained percentages of slow-wave sleep that exceed those recorded from controls [100]. Although the pathophysiology of idiopathic hypersomnia remains unknown, differences in slow-wave sleep between these patients and controls suggest involvement of the homeostatic regulation of sleep.
In patients with delayed sleep phase disorder, sleep onset and offset occurs later than desired [101]. Typical sleep onset is 2:00 AM to 6:00 AM and sleep offset is 10:00 AM to 2:00 PM [102]. The condition usually has been attributed to an abnormality in circadian timing, but a contribution from homeostatic factors also may be present. In comparison to controls, patients with delayed sleep phase disorder can demonstrate a prolonged time interval between the time of highest circadian propensity for sleep and sleep offset (abnormal phase angle) [103–105]. Additionally, these patients have increased amounts of slow-wave sleep in the latter portion of the sleep period, longer sleep durations, and impaired ability to recover during sleep deprivation [104, 106, 107]. Similar observations also have been made among sighted individuals with free-running circadian rhythm sleep disorder [108, 109]. Investigators hypothesize that difficulty accruing and dissipating homeostatic sleep pressure may be involved in the pathogenesis of delayed sleep phase disorder and free-running disorder in sighted individuals [106, 110]. The differences in homeostatic sleep regulation between morning and evening circadian phenotypes and the Per3 polymorphism discussed previously may further support this hypothesis. Furthermore, morning types (individuals who prefer earlier sleep wake times) demonstrate a larger buildup of slow-wave activity in response to increased sleep pressure and show a faster decline over the course of the night compared to evening types (individuals who prefer later sleep wake times) [111–115]. However, some studies of phase angle and sleep duration have not found differences between patients with delayed sleep phase disorder and normal controls [116]. Therefore, further research is needed to delineate the role of sleep homeostasis in circadian rhythm sleep disorders.
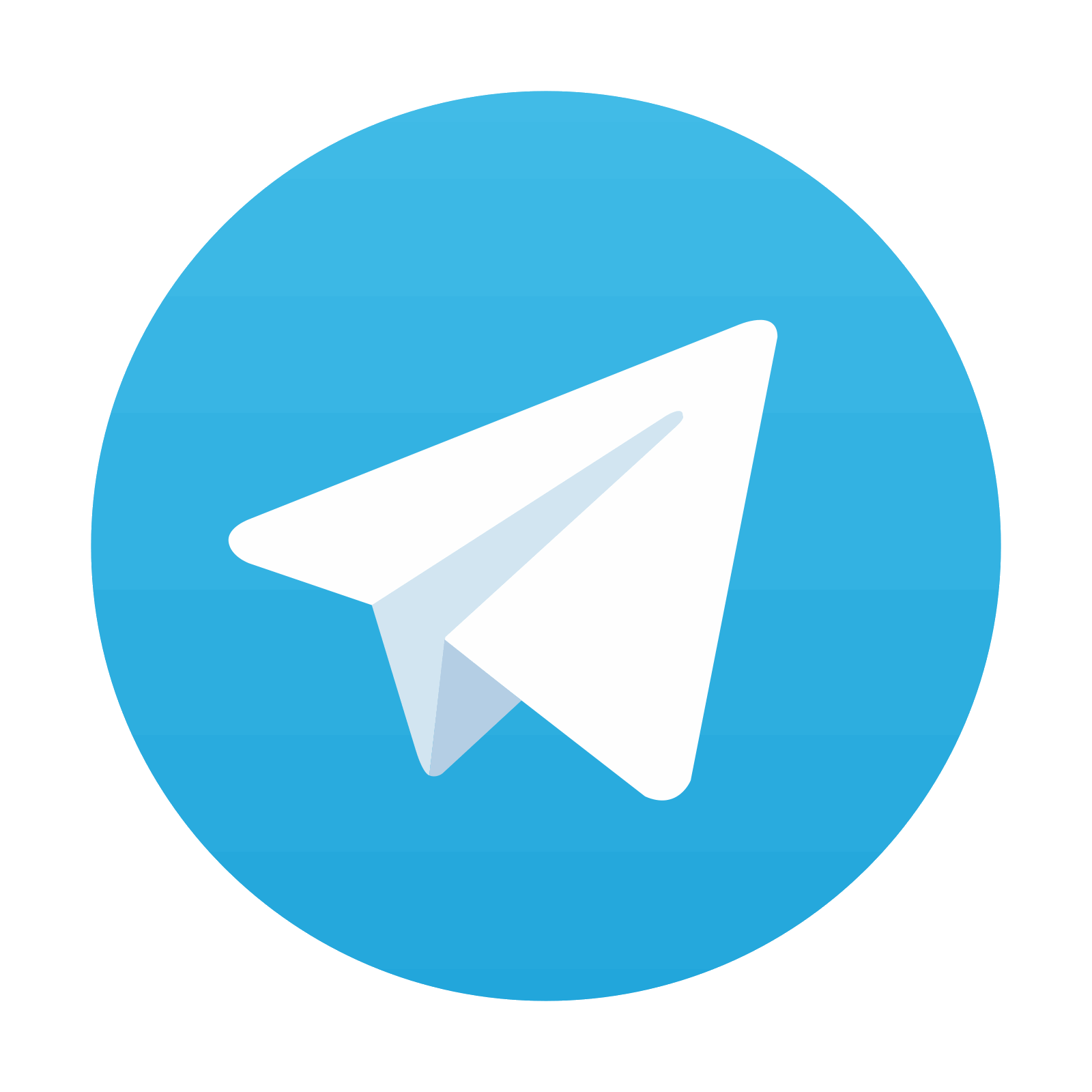
Stay updated, free articles. Join our Telegram channel
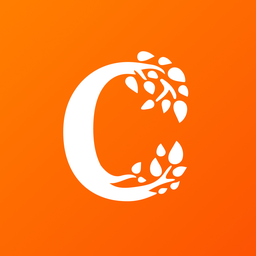
Full access? Get Clinical Tree
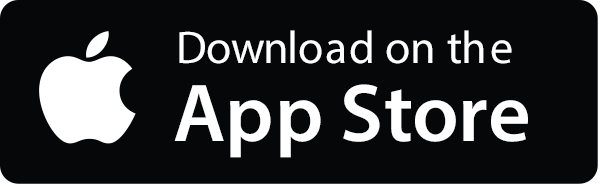
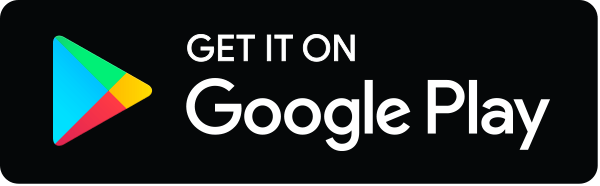