35 Radiosurgery and Radiotherapy for Benign and Malignant Anterior Skull Base Lesions Stereotactic radiosurgery (SRS) is the delivery of a single high dose of ionizing radiation to an intracranial target, which is defined by stereotactic image localization. The term stereotactic (derived from Greek “stereo,” meaning three dimensional [3D] and “tactos” meaning ordered or arranged) refers to techniques in which targets are precisely localized in three dimensions of space. Stereotactic localization allows for procedures to be performed such as surgery, biopsies, or the delivery of focused radiation. Ideal targets for SRS are small, round, discrete tumors with minimal microscopic spread or discrete functional targets. This allows the maximal target dose to achieve tumor control or functional ablation, while minimizing adjacent normal tissue irradiation. The term radiosurgery was first coined in 1951 by the neurosurgeon Lars Leksell.1 He is credited with the development of SRS for the treatment of functional disorders and tumors in the brain. Using the Leksell Gamma Knife (Elekta, Stockholm, Sweden) and linear accelerator (LINAC) radiosurgery units, such as the Radionics X-Knife (Integra Plainsboro, New Jersey), the SRS in the brain uses a rigid head frame externally fixed to the patient’s skull by a halo and pins with local anesthesia. The target localizer assembly fitted over the frame is used to determine the target coordinates, when used in conjunction with computed tomography (CT) and/or magnetic resonance imaging (MRI). In recent years, frameless SRS systems have been developed. These include the CyberKnife radiosurgery system (Accuray, Sunnyvale, California) pioneered by the Stanford neurosurgeon John Adler. Frameless SRS allowed the expansion of radiosurgery principles to extracranial sites without losing the accuracy of the stereotactic approach. Frameless SRS systems have also allowed greater flexibility in terms of radiation delivery using multiple treatments. Stereotactic radiotherapy (SRT) uses the same concept of radiation delivery as SRS. These, however, occur in multiple dose fractions usually using conventional daily dose fractionation schema of 1.8 to 2 Gy per fraction over 5 weeks, or hypofractionated SRT with higher doses delivered in two to five fractions. The decision to use SRS (single fraction) or hypofractionated SRT (2 to 5 fractions), instead of conventionally fractionated SRT is a function of the tumor size, tumor histology, and proximity to adjacent radiosensitive structures. Organs at risk such as the optic chiasm, optic nerves, and brainstem are of particular concern with anterior skull base lesions. The rationale for fractionating radiation treatments is to spare late responding normal tissue from long-term radiation toxicity. Tumors ≤ 3 cm in size are often amenable to single-dose SRS if there is adequate dose fall off to critical normal tissue. Larger tumors or tumors in very close proximity to a critical normal structure are usually amenable to hypofractionated or conventionally fractionated SRT. The overall objective of SRS and SRT is to achieve highly accurate treatment delivery and to maximize the therapeutic ratio of achieving tumor control while minimizing potential treatment-related toxicity to adjacent normal tissue at risk.2 SRS uses single fraction treatments ranging from 12 to 24 Gy depending on the disease and location. Fractionated radiosurgery uses hypofractionation over three to five fractions. Fractionated SRT uses conventional daily fractionated doses of 1.8 to 2 Gy delivered to the tumor typically over 5 to 6 weeks of treatment. In the skull base, the gross tumor volume (GTV3) is contoured on a high-resolution MRI which display the best set of images to accurately outline the GTV. Radiation works principally by inducing damage to the deoxyribonucleic acid (DNA) of cells either by direct or indirect damage. Direct damage occurs when a charged particle has sufficient energy to induce damage directly to an atomic structure. About two-thirds of damage to DNA is by indirect action from hydroxyl radicals generated from the radiolysis of water. When cells are irradiated, a single-strand or a double-strand DNA break can occur. Single-strand breaks can usually be repaired (sublethal repair), while double-strand breaks often result in the formation of unstable chromosomes (dicentric chromosomes) and therefore cell death via mitotic catastrophe. The efficacy of radiation and fractionation exploits the differential effect of radiation on tumor cells versus normal tissue. Cancer cells and normal nonmalignant cells are susceptible to radiation damage, but a therapeutic window exists in which radiation selectively damages cells with high mitotic rates. Tumors often have abnormalities in repair of single-stranded DNA breaks; this allows the differential effect between normal tissue repair and tumor cell kill. Cellular response to DNA damage centers on principles of cell cycle kinetics, cell cycle checkpoints (G1/S, intra-S phase, and G2/mitosis), and DNA repair of single-stranded and double-stranded breaks.4 Current models utilize 192 60Co sources that converge and focus onto a treatment target at a source to focus distance of approximately 40 cm. While each individual dose from the 192 sources is insignificant, the total convergent dose is therapeutically appropriate with minimal radiation to the surrounding normal tissues. The sources are aligned with the assistance of three collimator systems: the precollimator, the stationary collimator, and the final collimator (on the helmet). An appropriate helmet based on a circular collimator size (4, 8, and 16 mm) is chosen depending on the target size while the Leksell stereotactic frame is fixed to the patient’s skull. Heck et al reported the overall, long-term mechanical accuracy, and the precision of the Gamma Knife irradiated volume deviation to be –0.014 ± 0.09 mm, 0.013 ± 0.09 mm, and –0.002 ± 0.06 mm for the x, y, and z coordinates, respectively, with all measured data contained within a 0.2 mm sphere.5 Since its implementation approximately 30 years ago, over 400,000 patients have received treatment with the Gamma Knife SRS system. The long track record of the Gamma Knife unit coupled with 15-year follow-up clinical data ensures that the Gamma Knife device remains the benchmark and the current gold standard for intracranial radiosurgery.6–9 A frequently used system for the LINAC-based radiosurgery is the Radionics X-Knife.10,11 Since most modern LINACs use 6 MV photons, this is the most commonly used energy for LINAC-based radiosurgery. The system uses a series of noncoplanar arcs of circular beams converging at the mechanical isocenter around which the LINAC gantry, collimator, and treatment couch rotate. The physician places a rigid head frame on the patient’s skull and inserts four pins to prevent its movement relative to the skull. This frame is then mounted to the couch on the imaging device (CT and/or MRI). Once imaged, the frame is used to define the 3D Cartesian coordinate system for an accurate localization and irradiation of the target. Laser and imaging guidance are used to ensure the patient is positioned on the treatment couch, with the frame docked to the couch, such that the center of the planned treatment beams coincides with the nominal LINAC isocenter. If the head frame slips and the cranial anatomy is no longer at the same coordinates with reference to the head frame, then the process must be restarted. Lutz et al reported average errors of 1.3 ± 0.5 mm for CT imaging and 0.6 ± 0.2 mm for plane film radiograph.11 Localization errors with MRI were higher than that of CT.5 However, given better diagnostic abilities with MRI, it remains an integral part of SRS workflow. There have been no clinically significant differences between the Gamma Knife and the X-Knife reported in the literature.12 Another LINAC-based SRS can be used by retrofitting the outside LINAC head with micro multileaf collimators (mMLCs). Traditional multileaf collimators (MLCs) are contained inside the LINAC head and cast a projection of 5 or 10 mm at the LINAC isocenter depending on the make and model of the LINAC. These MLCs are used to shape radiation fields to conform around the GTV. The mMLCs are designed like the standard MLCs, except that the width of their tungsten leaves is considerably smaller providing a typical field width of 1.5 to 6 mm at the LINAC isocenter. This allows the mMLCs to shape the small radiosurgical fields with greater precision and to be used in static 3D SRS treatments, dynamic arc,13 and intensity-modulated radiation therapy.14–16 Usually for the LINAC-based SRS with mMLCs, only one isocenter is used for treatment planning.14,17–19 This design of SRS has considerable appeal because of the availability of multiple treatment techniques and planning options that are not limited by GTV size or geometry but still provide a comparable SRS and SRT option.20 An alternative approach to the head frame, the infrared optical-guided frameless system has been introduced. It consists of a customized mask and bite block with a rigid array of fiducial markers attached. A ceiling mounted infrared camera detects the array of infrared reflective markers, which in conjunction with the orthogonal kilovoltage images provides the patient setup guidance and intrafractional motion monitoring.21,22 Wang et al reported that 82% of the patients in their study had setup error of less than 1 mm.23 The CyberKnife was the first frameless robotic radiosurgery system for intracranial and extracranial tumors.24,25 A robotic arm with 6 degrees of freedom controls a lightweight 6 MV LINAC. The robotic arm can quickly reposition the LINAC and allows treatment to be delivered from many different angles without the limitations of traditional gantry systems. The CyberKnife system uses a tracking algorithm that computes the offset between live X-ray images and digitally reconstructed radiograph images by identifying and matching landmarks in the images. The landmarks depend on the tracking mode specified by the treatment plan. Different tracking modes include skull tracking, Xsight spine tracking, fiducial tracking, and Xsight lung tracking. Skull and Xsight spine trackings use the fixed relationship between skeletal anatomy and the tumor to identify the tumor throughout the treatment. Fiducial seeds implanted in or around the tumor before the treatment may be used for soft tissue targets, using the fiducial seeds as a reference point for tracking the tumor during treatment. Lung tracking uses a respiratory gating method that tracks the lung volume relative to the soft tissue anatomy to account for patient movement. The CyberKnife system uses repeated planar kilovoltage images throughout the treatment to monitor skeletal landmarks or fiducial markers, and adjusts the robotic arm position accordingly. Typically, 100 to 200 static beams are delivered through fixed collimators for CyberKnife radiosurgery. The “Iris” is a variable aperture collimator recently developed to allow for multiple collimator sizes during a given treatment, without requiring change in collimator attachment during treatment. The reported accuracy of the CyberKnife system is 1.1 ± 0.3 mm.26 Dosimetric studies have reported excellent tumor dose distribution with the CyberKnife system.27,28 The first publication theorizing the therapeutic use of protons was published in 1946 by Robert Wilson.29 Charged particle beam radiosurgery produced by cyclotrons or synchrotrons creates a highly focused dose distribution by virtue of the Bragg peak effect; this deposits radiation over a specified range with minimal exit radiation dose. Hospital-based cyclotrons with high beam energies are able to treat deep-seated tumors, thus making protons a treatment of choice for skull base tumors. Tumors in close proximity to the brain, brainstem, spinal cord, optic chiasm, and cochlea can be treated with protons with sharp dose gradients between tumor and adjacent critical structures and thereby minimizing potential long-term treatment-related morbidity.30 Another benefit of proton treatment is substantially reduced integral dose (by factor of 2) as compared with photon treatment.31 This makes proton treatment particularly beneficial for pediatric patients to reduce dose to developing organs and skeletal structures to minimize risk of growth delay. Proton facilities typically use a passive scattering beam delivery method in which the primary proton beam is degraded with scattering foils. A range modulator spreads out the Bragg peak into a clinically useful beam. Additional beam shaping can then be achieved by using patient-specific devices including brass apertures, which are made to shape a specific field to achieve lateral field confirmation and a polymethyl methacrylate range compensator to achieve distal confirmation. Newer techniques include active scanning method in which the proton beam is delivered by a spot or narrow pencil beam and the dose is deposited in layers. With this technique, the beam can also be modulated using intensity-modulated proton beam therapy, in which irregularly shaped tumors can be treated with very high conformity.32 Treatment planning relies on reconstruction of axial CT imaging of the patient in the treatment position, commonly referred to as radiation simulation. A virtual patient is created by the treatment planning software by importing the CT scan, and often fusing it with adjunct imaging studies. Most commonly in the skull base, MRI is used to assist target delineation. This allows the physician to delineate both tumor targets and adjacent normal organ structures.33 The radiation physicist or dosimetrist programs the software to determine the optimal beam angles, beam weights, and collimation to achieve the dose objectives set by the physician. The radiation dose distribution can be seen on 2D or 3D reconstructed views from the CT images obtained at the time of radiation simulation. A dose volume histogram (DVH) is generated to determine the percentage of the volume encompassed by the percent of the total dose. The DVH only gives information about the volume of the target as a whole and not necessarily information about the anatomic location of variations in the dose.34 Typically in Gamma Knife radiosurgery, the prescription dose is delivered to the 50% isodose line encompassing the target volume (TV), with a central maximum dose being twice that of the prescription dose. In LINAC-based radiosurgery, the prescription dose is often prescribed to higher isodose lines, usually 80%, and hence the maximum dose is less than that delivered by Gamma Knife. Conformity indices (CIs) are used to compare competing plans, evaluate treatment techniques, and assess the risk of potential complications.27 The CI is defined as the ratio of prescription volume (PV) to TV, and usually ranges from 2.7 for conventional LINAC radiosurgery to 1.8 for mMLC collimator radiosurgery.35,36 The new conformity index (nCI) used by the University of California, San Francisco, group includes calculating the ratio of the TV within the prescribed isodose surface (TVPV) to the total TV, and dividing the ratio of PV to TVPV by the coverage (to account for the location of the PV with respect to the TV) as devised by Nakamura et al37 and Paddick.38 Hence, an ideal plan would have a TVPV = TV = PV, yielding an CI = 1.0 as well as a CI = 1.0. Once the plan is evaluated by these various quantitative and qualitative measures by the physician, the plan is then digitally transferred to the treatment machine. Consideration of dose to normal tissue in the skull base is important to reduce long-term complications of radiosurgery. In a series of 50 patients treated with SRS for benign skull base tumors, the incidence of optic neuropathy was zero for patients who received a radiation dose of < 10 Gy, 26.7% for patients receiving a dose in the range of 10 to < 15 Gy, and 77.8% for those who received doses of 15 Gy or more (p< 0.0001).39 Previously impaired vision improved in 25.8% and was unchanged in 51.5% of patients. No signs of cranial neuropathy were seen in patients in whom the cranial nerves of the cavernous sinus received doses between 5 and 30 Gy. Tumor control was achieved in 98% of patients. This study demonstrated that structures of the visual pathways (i.e., the optic nerve, chiasm, and tract) exhibit a much higher sensitivity to single-fraction radiation than other cranial nerves. In contrast, the oculomotor and trigeminal nerves were noted to have a higher radiation dose tolerance.39 For the Gamma Knife system, the stereotactic head frame is attached to the treatment couch at a correct position and docking angle before treatment. During treatment, the couch is moved so that each shot is delivered at the center of the focusing helmet. For conventional LINAC-based radiosurgery, the stereotactic head frame is docked to the treatment couch such that the center of each treatment arc coincides with the nominal LINAC isocenter. For optical-guided frameless LINAC-based radiosurgery, the patient is immobilized with the thermoplastic mask and custom bite block with the infrared reflective markers. During the treatment, both the infrared camera and the kilovoltage images are guiding the robotic couch motion depending on the patient deviation from the baseline position. During CyberKnife treatment, the patient is immobilized with a custom thermoplastic mask for skull base tumors and lies on the robotic couch (before treatment), while the stereo-imaging system tracks the patient in real time and adjusts the robotic couch and robotic arm position (during treatment) accordingly. All radiation machines have a record and verify system to ensure accuracy and to eliminate potential errors of treatment delivery.34 Radiosurgery is an important treatment modality for the management of skull base tumors, including meningiomas, pituitary adenomas, glomus tumors, and chordomas. Emerging applications of radiosurgery in the treatment of other skull base malignancies include nasopharyngeal cancer and those who have failed previous conventional external beam radiotherapy. Meningiomas, which arise from arachnoid cap cells, are benign, slow-growing, well-circumscribed solid tumors.40 Meningiomas account for 30% of adult intracranial neoplasms with incidence increasing with age, peaking in the 5th or 6th decade, and a 2:1 female predilection.40 A history of previous radiation exposure has also been reported to increase ones risk. Symptoms at presentation vary widely as they largely reflect tumor location, with common locations including cerebral convexities, falx cerebri, tentorium cerebelli, cerebellopontine angle, sphenoid ridge, and spine. These tumors may undergo malignant transformation, can be locally destructive, and cause adjacent bony erosion or hyperostosis. Meningiomas are graded according to World Health Organization (WHO) grading system, most of which are benign or WHO grade I, but approximately 35% of all meningiomas are atypical (WHO grade II) or malignant (WHO grade III).41 Therapeutic options are varied, ranging from observation with serial imaging, surgery, radiosurgery, or fractionated external beam radiotherapy.41 Determinants of management include the character of the meningioma by imaging (benign versus atypical or malignant), rate of progression on imaging, tumor size, and the presence or absence of surrounding edema. Asymptomatic, small, benign lesions without any mass effect are often reasonable to follow with observation. Tumors < 3 cm in dimension can be considered for curative SRS. The treatment of choice for symptomatic or progressive benign meningiomas > 3 cm is complete surgical resection. However, meningiomas of the optic nerve sheath42–44 and skull base tumors located in the petroclival area and cavernous sinus can be primarily treated with radiation therapy, with SRS,45–55 hypofractionated SRT,47 or conventionally fractionated SRT56–59 with excellent functional outcome and local control. Often tumors in these locations are not amenable to surgery without significant morbidity. The decision to use single fraction SRS or fractionated radiation treatment depends on the proximity and potential radiation dose to the adjacent critical organs at risk such as the optic nerves and chiasm. Fig. 35.1 shows a treatment plan for a left cavernous sinus meningioma. Outcome data for radiation treatment have been reported in several studies. Flickinger et al from the University of Pittsburgh reported on 219 meningiomas, which underwent Gamma Knife radiosurgery to a median marginal tumor dose of 14 Gy with a median follow-up of 29 months.60 The actuarial 10-year tumor control rate was 93.2% and the 10-year actuarial rate of developing any postradiosurgical injury was 8.8%. Similarly, an European study evaluated 331 patients with 356 meningiomas treated with Gamma Knife radiosurgery to a median tumor marginal dose of 12.55 Gy. The 5-year actuarial tumor control rate was 97.9%.61 The overall treatment morbidity was 10.2% with a permanent morbidity rate of 5.7%, and a treatment-specific mortality rate of 0.6%. Tumors > 10 cm3, peritumoral edema, and meningiomas in the anterior skull base were reported to be at higher risk of posttreatment complications. The University of Maryland identified factors independently associated with treatment outcomes, on multivariate analysis, after Gamma Knife SRS and reported that patients with a GTV > 10 cm3 were more likely to have tumor recurrence (hazard ratio, 4.58; p = 0.05).62 Additionally, patients who had lower CIs with improved coverage of the dural tail had better control rates on univariate analysis. Similar outcome data for CyberKnife have been reported. A University of Pittsburgh study reported on the efficacy of CyberKnife in a cohort of 73 patients (60 WHO grade I, 11 WHO grade II, and 2 WHO grade III).63 The actuarial 1-year local control rate was 95, 71, and 0% for WHO grades I, II, and III, respectively, when treated to a median dose of 17.5 Gy over a median of three fractions. A subjective improvement in existing tumor-related symptoms was noted in 60% of patients. In a series of 199 meningiomas, predominantly of the skull base, the 5-year local actuarial control rate was 93.5% after CyberKnife radiosurgery to a mean dose of 18.5 Gy.47
Current Radiosurgery Techniques
Gamma Knife Stereotactic Radiosurgery
LINAC-Based Stereotactic Radiosurgery with Circular Cone Collimation
LINAC-Based Stereotactic Radiosurgery with Micro Multileaf Collimators
CyberKnife Stereotactic Radiosurgery
Proton Stereotactic Radiosurgery
Treatment Planning and Dosimetry
Treatment Execution
Clinical Sites
Meningiomas
Stay updated, free articles. Join our Telegram channel
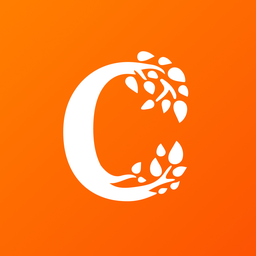
Full access? Get Clinical Tree
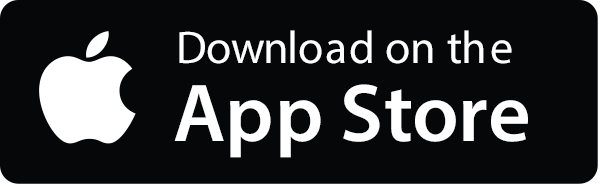
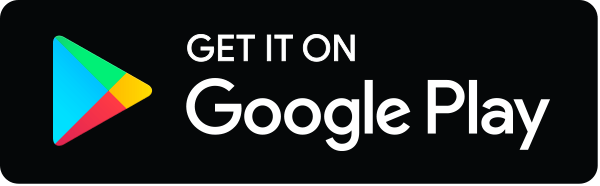