, Maneli Mozaffarieh1 and Hans Bebie2
(1)
Department of Ophthalmology, University of Basel, Basel, Switzerland
(2)
Institute for Theoretical Physics, University of Bern, Bern, Switzerland
Abstract
The word “protein” is derived from the Greek word “protos,” meaning “of prime importance.” Proteins are involved in a variety of functions, all of which are essential to life. Proteins give strength and elasticity to skin and blood vessels. In the blood, they serve as protectors in the form of antibodies or as long-distance transporters of oxygen and lipids; in the nervous system, they form part of the communications network by acting as neurotransmitters; and throughout the body, they direct the work of repair, construction, and energy conversion.
The word “protein” is derived from the Greek word “protos,” meaning “of prime importance.” Proteins are involved in a variety of functions, all of which are essential to life. Proteins give strength and elasticity to skin and blood vessels. In the blood, they serve as protectors in the form of antibodies or as long-distance transporters of oxygen and lipids; in the nervous system, they form part of the communications network by acting as neurotransmitters; and throughout the body, they direct the work of repair, construction, and energy conversion.
The role of proteins in ophthalmology will be discussed in this section. First, however, we will briefly discuss how proteins were discovered in the first place.
16.1 Discovery of Proteins
Proteins were originally described in the early eighteenth century by the Dutch chemist Mulder1 and the Swedish chemist Berzelius.2 Mulder was the first person to ascertain the chemical composition of proteins and he observed that all proteins had the same empirical formula. At the time, his colleague Berzelius was the first to distinguish between organic and inorganic compounds. Berzelius proposed the name “protein” for Mulder’s new findings and Mulder, in fact, used this terminology in his first publication.
Later, the German chemist and physiologist von Voit3 stated that protein is the most important nutrient in the structure of the body. Voit was considered by many to be the father of modern dietetics. The enzymatic role of proteins was first discovered in the nineteenth century by Sumner,4 who showed that the enzyme urease is a protein. Some years later, the first protein sequence, that of insulin, was presented by Sanger,5 who won the Nobel Prize for this achievement in 1958. In the same year, the molecular structures of the proteins hemoglobin and myoglobin were discovered by Max Perutz6 and Kendrew.7
Emil Fischer8 (Fig. 16.1) proposed that enzymes had particular shapes into which substrates fit exactly. He first postulated in 1894 this specific action of an enzyme with a single substrate.
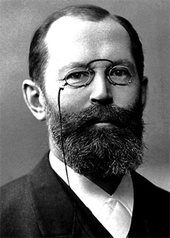
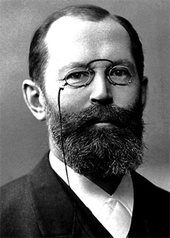
Fig. 16.1
Emil Fischer
This model of exact fit is referred to as the “lock and key” model because an enzyme’s binding to a substrate is analogous to the specific fit of a key into a lock (Fig. 16.2).
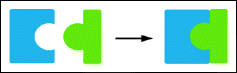
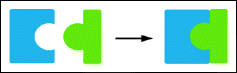
Fig. 16.2
Lock and key model. Only the correctly sized key (substrate) fits into the keyhole (active site) of the lock (enzyme)
16.2 Structure of Proteins
The primary structure refers to the linear arrangement of amino acids (Fig. 16.3).
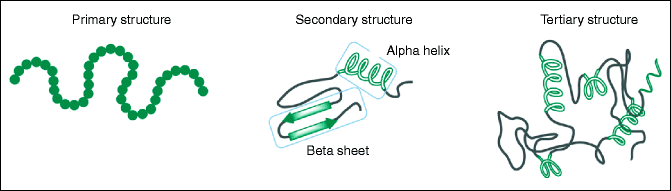
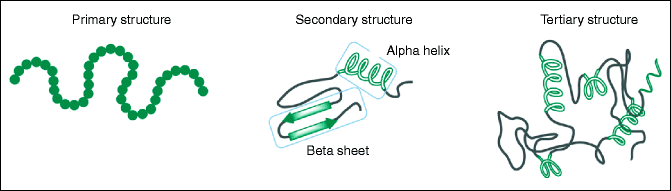
Fig. 16.3
Different structures of proteins
The sequence of amino acids determines the function of the protein. Variations in the amino acid sequence may occur physiologically, such as in polymorphism, which occurs when two or more clearly different phenotypes exist in the same population of a species – in other words, more than one form or “morph” occurs. Polymorphism allows for diversity within a population. A common example is the different allelic forms that give rise to different blood groups in humans.
However, variations in the amino acid sequence may also occur in the form of mutations. Mutations can result in several different types of changes in the amino acid sequence. These can have no effect or they may prevent the protein from functioning properly at all. Retinitis pigmentosa (Fig. 16.4) is one example of a genetic group of eye disorders that results from mutations. Multiple genes are known that, when mutated, can cause the retinitis pigmentosa phenotype. In 1989, a mutation was identified in the gene for rhodopsin (Sect. 16.5.4). Since then, more than 100 other mutations leading to RP have been found.
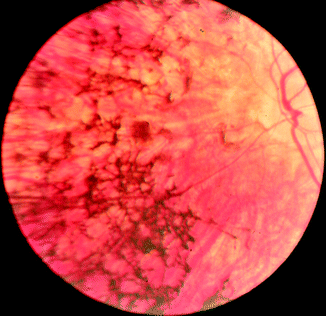
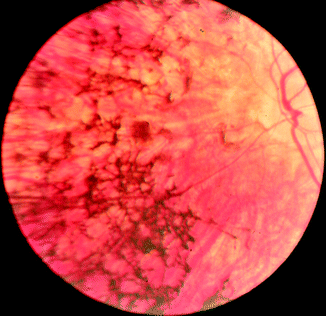
Fig. 16.4
Retinitis pigmentosa. Fundus of patient with retinitis pigmentosa. Bone spicule-shaped pigment deposits are present in the mid-periphery, together with retinal atrophy
Corneal dystrophies are another example of eye disorders resulting from mutations. Some corneal dystrophies may be asymptomatic, whereas others may cause significant vision impairment. An example is type 1 lattice dystrophy, one of the most common inherited corneal dystrophies, which is characterized by the accumulation of amyloid throughout the middle and anterior stroma. Type I lattice dystrophy is an autosomal dominant disorder that results from mutations in the TGFBI gene (5q31). The TGFB1 gene was first discovered in 1997 by Munier et al. in Lausanne, Switzerland. This gene encodes a member of the transforming growth factor beta (TGFB) family of cytokines, which are multifunctional peptides that regulate proliferation, differentiation, adhesion, migration, and other functions in many cell types. Many cells have TGFB receptors and the protein positively and negatively regulates many other growth factors. Mutations in the TGFB-1 gene can lead to various pathologies, including corneal dystrophies (Fig. 16.5).
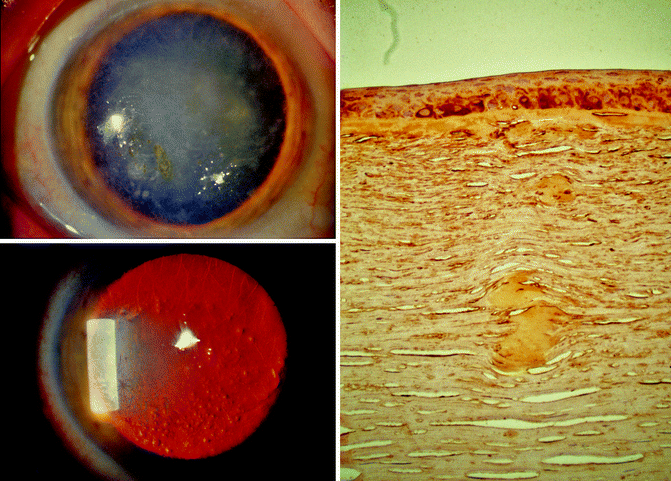
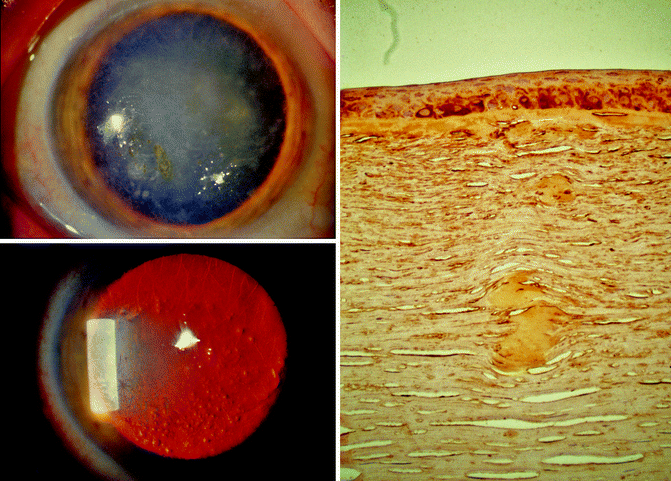
Fig. 16.5
Left: biomicroscopic views under diffuse light (top) and retroillumination (bottom) showing a lattice type 1 corneal dystrophy linked to a R124C heterozygote mutation in the TGFBI gene. Right: light microscopy from a lattice type 1 patient (TGFBI R124C) with kerato-epithelin immunostaining showing subepithelial and stromal amyloid deposits. (From Munier FL, et al. (1997) Nat Genet, 15. With permission)
Another example of a disorder resulting from mutations is galactosemia. Several types of galactosemias are recognized, each of which is caused by mutations in a particular gene and affect different enzymes involved in breaking down the sugar galactose. Infants with galactosemia who are not treated promptly with a low-galactose diet face complications. Affected children are also at increased risk of delayed development, speech difficulties, and intellectual disability, as well as the development of lens cataracts.
Lens dislocations that occur in the context of Marfan syndrome (Fig. 16.6) are often due to a mutation in the fibrillin-1 molecule. Fibrillin-1 is an extracellular matrix glycoprotein protein that is the main component of the microfibrils. Multiple autosomal dominant mutations in FBN1 have been described for a wide spectrum of disease, including complete and incomplete forms of Marfan syndrome.
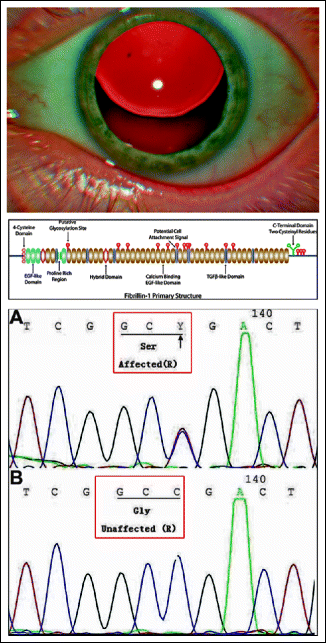
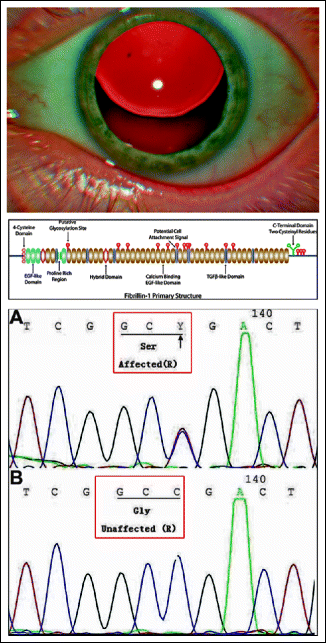
Fig. 16.6
Marfan syndrome. Top: subluxated lens. Middle: fibrillin-1 is an extracellular matrix glycoprotein. Different mutations of this protein can lead to Marfan syndrome. Bottom: example of a fibrillin mutation resulting in a glycine-to-serine exchange. (Middle: from Baylor College of Medicine, Department of Pediatric/Cardiology, Houston/Texas. With permission. Bottom: from Dong J, et al. (2012) MolVis 18. With permission)
The secondary structure describes the folding of the polypeptide backbone of the protein (Fig. 16.3). Two main types of secondary structures are recognized: the alpha helix and the beta sheet. The alpha helix takes the form of a spiral structure consisting of a tightly packed, coiled polypeptide backbone core with the side chains of the amino acids extending outward from the central axis. The keratins, for example, are a family of fibrous proteins whose structure is nearly entirely alpha-helical. The beta sheet is another secondary structure in which all of the peptide bond components are involved in hydrogen bonding. The surfaces of these beta sheets appear to be “pleated,” which is why they are often called “beta-pleated sheets.”
The third type of structure found in proteins is called the tertiary structure (Fig. 16.3). The tertiary structure is the final specific geometric shape that a protein assumes. This final shape is determined by a variety of bonding interactions between the side chains on the amino acids. These types of bonding interactions include hydrogen bonding, salt bridges, bisulfate bonds, and nonpolar hydrophobic interactions. Disulfide bonds, for example, occur between the sulfurs of two cysteine side chains. An example of a protein with many disulfide linkages between cysteines is found physiologically in the lens zonules. Therefore, a cysteine deficiency may affect normal zonular development (the zonules become brittle and can break), leading to lens dislocation.
The quaternary structure of a protein is a larger assembly of several protein molecules or polypeptide chains. As in the tertiary structure, the quaternary structure is stabilized by noncovalent interactions (e.g., hydrogen bonds or ionic bonds). Complexes of two or more polypeptides are called multimers (e.g., a protein is a tetramer if it contains four subunits). These subunits may either function independently of each other or work cooperatively, as in the case of hemoglobin, where the binding of oxygen to one subunit of the tetramer increases the affinity of the other subunits for oxygen (Fig. 16.7).
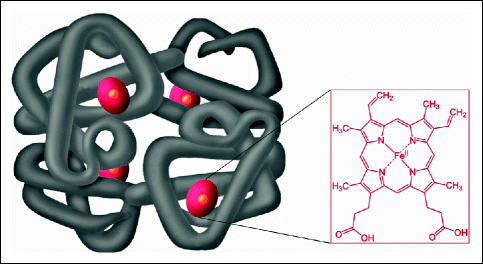
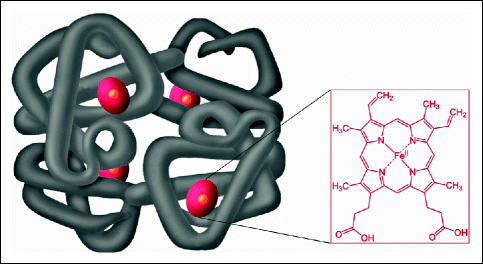
Fig. 16.7
Hemoglobin. The iron-containing heme groups in red are embedded in a protein that consists of two α and two β subunits
16.3 Information Content of a Protein
All proteins are synthesized from only twenty naturally occurring amino acids, which belong to the group of α-amino acids (one carbon, the α-carbon, has all of the remaining groups attached to it). These groups include an amino group, a carboxyl (acidic) group, hydrogen, and one of twenty different R groups (side chains) that give each amino acid type its specific properties. Proteins are polypeptides; i.e., they are single linear chains of amino acids bonded together by peptide bonds (Fig. 16.8).
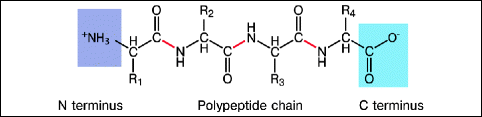
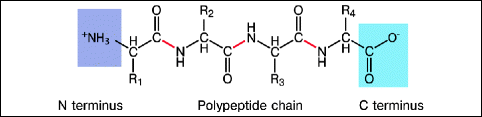
Fig. 16.8
Polypeptide chain. In a polypeptide chain, the amino acids are bound together by peptide bonds (red)
We shall first discuss the role of proteins in general and then in the eye.
16.4 Roles of Proteins
Proteins are the most common and manifold macromolecules in living cells. They are found in all cells. One single cell contains thousands of different proteins whose biological functions may differ greatly from each other. Proteins occur also outside the cells. The proteins in the blood, for example, serve as protectors (e.g., in the form of antibodies) or as long-distance haulers of molecules such as oxygen (e.g., hemoglobin). Some proteins function as structural proteins that confer stiffness and rigidity to otherwise-fluid biological components. Other proteins, such as signaling proteins, form a part of the communications network of our nervous system. Certain proteins can also function as “chaperones” and assist in the folding, unfolding, and assembly of other proteins. The central role of all proteins, however – as we will show later – is their role as the most important end-products of information flow between and within cells. In a certain sense, proteins are the molecular instruments through which genetic information is expressed.
When a protein must be available at short notice or in large quantities, it is produced in its inactive form, called a pro-protein (e.g., proinsulin). This inactive form of the protein consists of a long chain of amino acids as well as one or more inhibitory peptides that can be activated when the inhibitory sequence is removed. This long chain of amino acids directs the transport of proteins to their specific location (e.g., insertion into membranes, as in the case of secretory proteins). A part of this long chain of amino acids, thus, acts as an “address.” Once the protein has arrived at the specific location, these sequences of amino acids can be removed or processed. Further splicing can activate the protein.
The activity of other proteins and of enzymes in particular can be regulated by the addition or removal of phosphate groups. Protein phosphorylation (the addition of a phosphate group) is recognized as one of the primary ways in which cellular processes are regulated. Phosphorylation reactions are catalyzed by a family of enzymes called protein kinases that utilize ATP as a phosphate donor (Fig. 16.9).
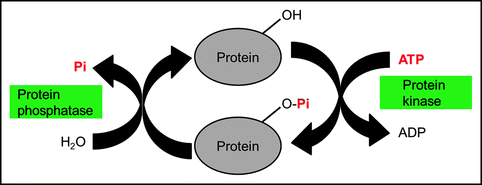
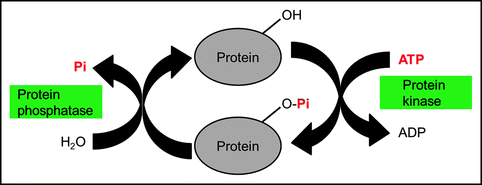
Fig. 16.9
Activation of proteins. Phosphorylation activates (or sometimes deactivates) proteins and dephosphorylation deactivates them
Phosphate groups are removed from phosphorylated enzymes by the action of phosphoprotein phosphatases. Depending on the specific enzyme, the phosphorylated form may be more or less active than the unphosphorylated enzyme. The discovery of reversible protein phosphorylation was made by the biochemists Fischer and Krebs9 (Fig. 16.10).
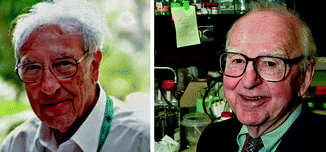
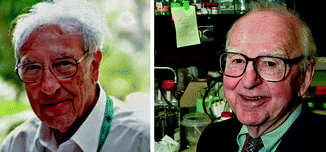
Fig. 16.10
Edmond Fischer and Edwin G. Krebs
Proteins can be grossly classified into those with functional and those with structural properties. We will not focus on the classification of proteins in any further detail but will, instead, concentrate on proteins that play a special role in the eye.
The overall role of proteins in the eye is principally the same as in all other tissues in the body. However, there are a few peculiarities.
16.5 Roles of Proteins in the Eye
16.5.1 Proteins in the Cornea
It may be surprising that the transparent cornea consists mainly of macromolecules, in addition to water. The volume of the cornea comprises mainly of extracellular matrix, which, in turn, comprises macromolecules and water. The cornea provides a good example of the role of structural proteins. The transparency of the cornea is made possible by the spatial arrangement of its structural proteins. These proteins, therefore, maintain stability but, as a result of their alignment, they also allow light to pass through the cornea (Sect. 2.3). In contrast, the sclera scatters all wavelengths of light and, therefore, appears white. This is because the collagen fibers in the sclera are not aligned in a parallel array, so they scatter light.
Under physiological conditions, “cross-linking” occurs between the parallel-running collagen fibers of the cornea (Fig. 16.11).
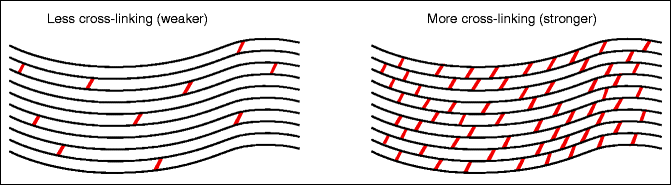
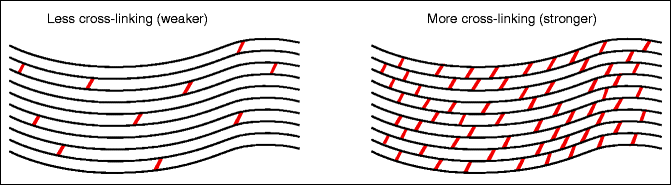
Fig. 16.11
Corneal stability. In the cornea, the fibers are linked together (cross-linking). These links can be weaker (left) or stronger (right)
Under physiological conditions, this cross-linking is achieved by the formation of covalent bonds (Fig. 16.12), which gives the cornea its characteristic stability and stiffness.
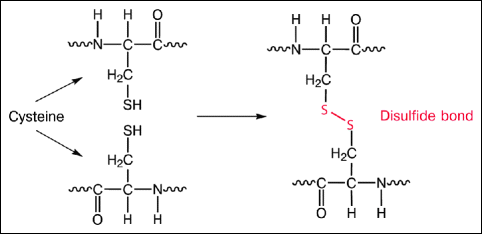
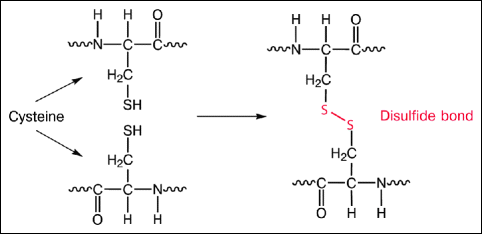
Fig. 16.12
Cross-linking. Cross-linking leads to the formation of covalent bonds (disulfide bonds) between the collagen molecules
In certain pathological conditions (e.g., keratoconus) or after laser treatment (e.g., Lasik), the natural covalent bonds may not be strong enough to prevent the parallel-running collagen fibers from sliding against each other. This risk is reduced by introducing an iatrogenic cross-linking, which leads to the formation of additional bonds between the collagen molecules (Fig. 16.13).
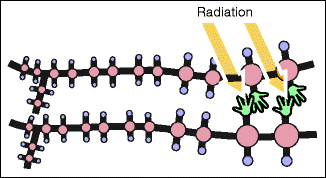
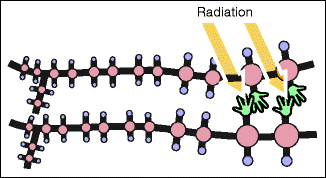
Fig. 16.13
Therapeutic cross-linking. Additional cross-linking can be achieved by the radiation of artificially added riboflavin, which acts as a photosensitizer
This is achieved by induced oxidative stress: after abrasion of the corneal epithelium, eye drops containing riboflavin (a photosensitizer) are applied to the eye (Fig. 16.14). The eye is then illuminated by UV light (365 nm). This activates the riboflavin molecule, which can then transfer energy to a nearby oxygen molecule, thereby turning ground-state oxygen into the highly active singlet oxygen. Singlet oxygen then transfers this energy to form covalent bonds. However, the exact type of chemical bond that is induced is still not yet clear.
< div class='tao-gold-member'>
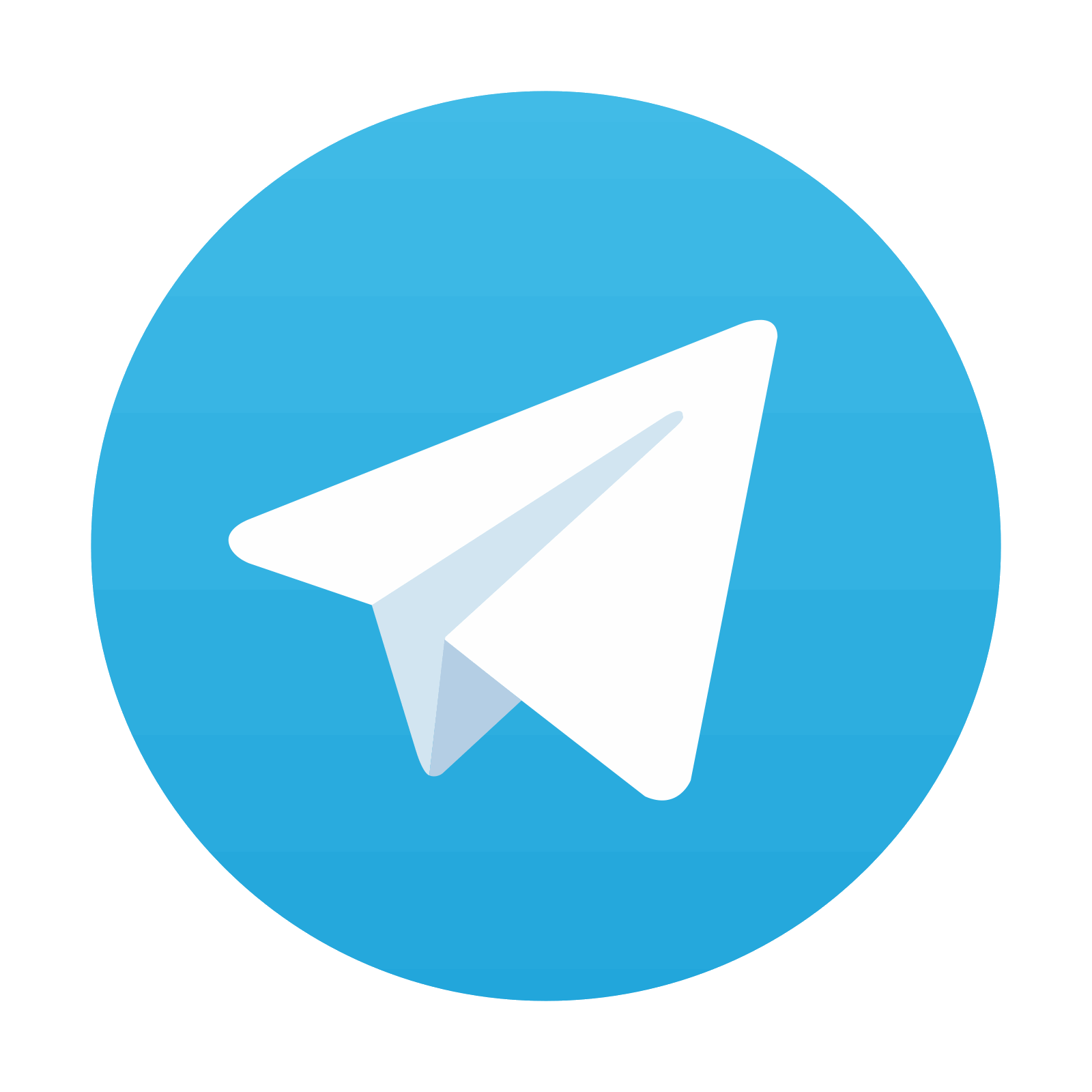
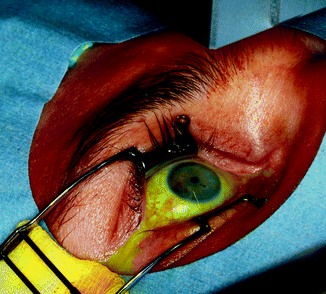
Only gold members can continue reading. Log In or Register a > to continue
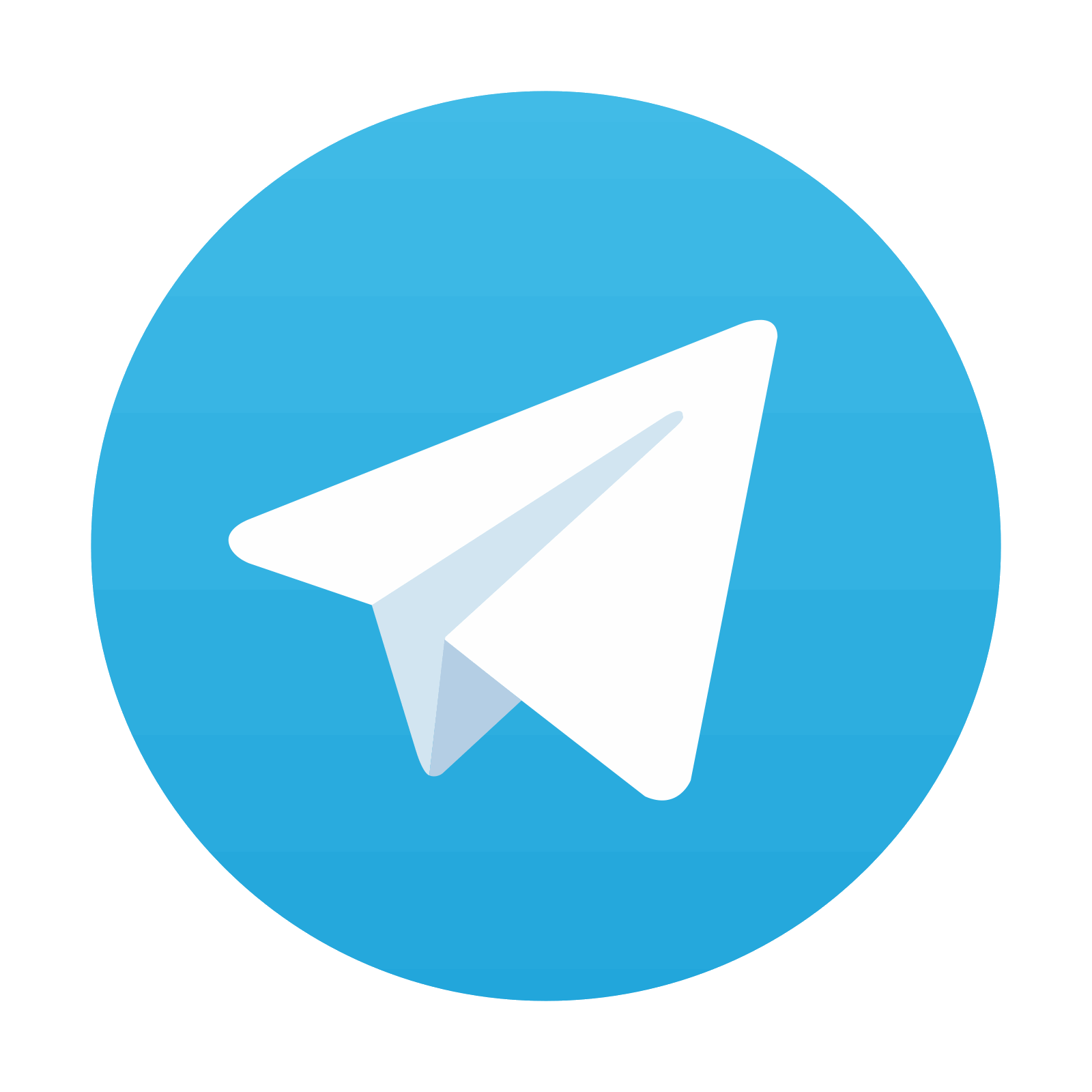
Stay updated, free articles. Join our Telegram channel
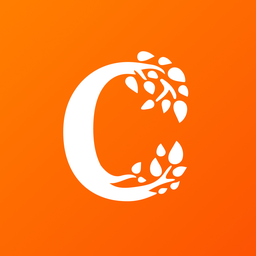
Full access? Get Clinical Tree
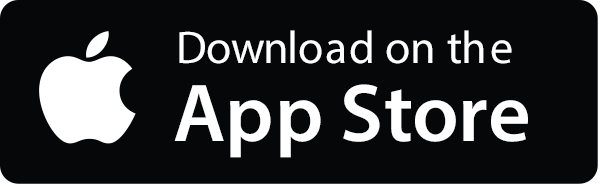
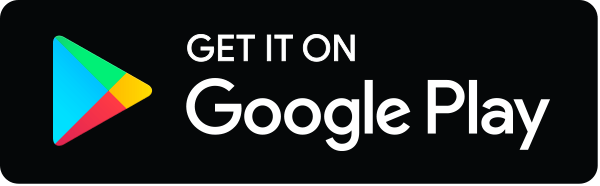
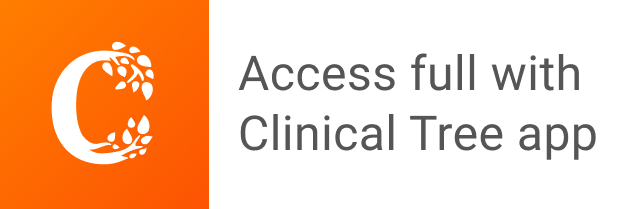