Obstructive sleep apnea (OSA) results from complex interactions between anatomy and physiology. A structurally small and abnormally collapsible upper airway predisposes to disease, and interacts with normal and pathologic physiologic mechanisms to determine severity of disease. Understanding the pathophysiology provides insight into airway collapse, and may improve treatment and lead to potential new medical and surgical treatments for OSA.
Obstructive sleep apnea (OSA) is a common disorder resulting from collapse of the pharyngeal airway during sleep. The cause and mechanisms of this collapse are multifactorial, but ultimately, it results from the interdependence of structurally vulnerable upper airway anatomy interacting with physiologic mechanisms of ventilatory instability and reduced or absent dilator muscle control in the sleep state. Our understanding of the pathophysiology of OSA has evolved over the last several decades. Historically, two basic schools of thought existed to describe the genesis of airway collapse: “active” versus “passive” mechanisms. The active theory proposed by Weitzman and colleagues in 1978 resulted from their observation of spasmodic closure of the lateral pharyngeal walls and closure of the velopharynx timed at the end of expiration in animal models. This sphincteric closure “apparently by active muscle contraction” was maintained for the duration of inspiration, and was followed by airway opening occurring following arousal from sleep. Because electromyographic studies of pharyngeal constrictors in humans fail to demonstrate expiratory muscle activity during airway collapse, the concept of active muscular contraction causing airway closure in OSA has been abandoned in favor of other explanatory mechanisms . An alternative to the active theory is based on passive mechanisms that do not require active neuromuscular contraction of pharyngeal muscle to close the airway. Airway obstruction instead results from the interaction of loss of dilating activity of pharyngeal muscles during sleep, as well as the mass of the tongue and other tissues, and their interplay with inspiratory intraluminal pressures . As early as 1978, Harper and Sauerland observed a loss of both phasic and tonic electromyographic tongue activity during non-rapid eye movement (NREM) sleep in OSA subjects. This loss of activity was not seen in snoring subjects (is this then a compensatory mechanism in snoring that protects them from obstruction?). Both OSA and snoring subjects contrasted with normal nonsnoring individuals. Normal subjects did not have significant electromyographic activity in the genioglossus muscle during wakefulness or NREM sleep. Their findings suggested that muscle activity was important in maintaining stability in OSA subjects, but that loss of muscle activity alone was inadequate to create airway closure. Additional structural features such as a “large tongue” contributed to collapse, which when combined with negative inspiratory airway pressures, obstructed the airway. One could thus conclude that if the subject has a structurally compromised airway anatomy, he will suffer from OSA; however, this is not always the case. There appears to be a synergistic impact of the anatomy and the ventilatory control stability. Jordan and colleagues have provided evidence to explain the development of OSA through ventilatory instability in the OSA population with a loop gain model has been described . These concepts of structural compromise interacting with nervous system response to ventilatory disturbance form the current working model of OSA physiology.
The human upper airway has a complex task. It has the constant challenge of maintaining ventilation, while simultaneously allowing for alimentation, phonation, and speech. In other mammals, the larynx is positioned near the skull base, creating a physiologically separate respiratory and alimentary pathway . Anatomically, the tongue is an oral structure. The intimate relationship to the skull base results in a highly stable airway that is independent of muscle tone. In adult humans, however, the larynx resides in the neck, separated from the bony enclosure of the craniofacial skeleton ( Fig. 1 ). The tongue is oral and pharyngeal, and is more critical in supporting ventilation. This results in a longer pharyngeal airway, which further increases with age, male gender, and OSA. The laryngeal descent process (klinoraphy), while facilitating speech development, predisposes the soft tissue supra-glottic pharyngeal airway to obstruction and requires compensatory mechanisms to maintain stability . In such a setting, the addition of otherwise nonpathologic structural or physiologic changes may lead to upper airway collapse during sleep.
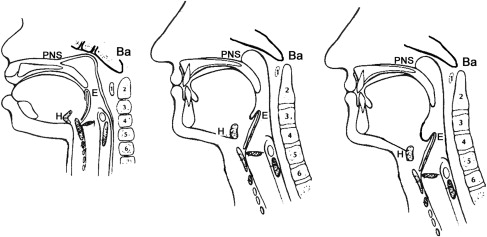
Multiple mechanisms are intricately involved to maintain upper airway stability. Anatomical structure, neuromuscular tone, ventilatory control mechanisms, level of consciousness (sleep and arousal effects), upper airway reflexes, peripheral nervous system mechanisms, craniofacial and soft tissue structure, body position, vascular tone, surface tension forces, lung volume effects, and expiratory collapse may all contribute to upper airway stability.
Structural considerations: anatomy abnormalities
Structure significantly predisposes to development, and is a fundamental abnormality of OSA . Structure effects of airway size, compliance, and shape are all critical to flow. Although the pharynx is the core, no single morphologic abnormality exists ( Fig. 2 ) that explains all OSA. The three major structural determinates are obesity, soft tissue, and skeletal morphology . In addition, structures associated with sleep disordered breathing may also be impacted by the additional factors of ethnicity, gender, obesity, and age .
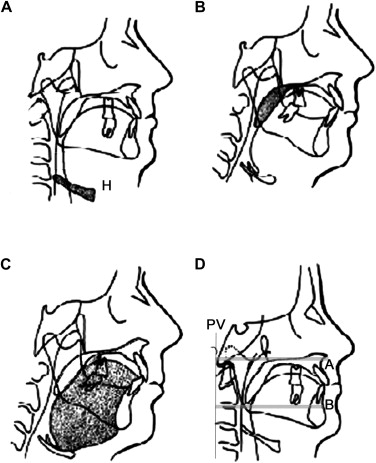
In children, adenotonsillar hypertrophy predominates as a cause. This condition is not unique to children, and other craniofacial and soft tissue abnormalities also are present in childhood . In fact, these other abnormalities may be the main predisposing factors leading to obstruction, and adenotonsillar hypertrophy may simply weight the scales further toward OSA.
In adults, no single structural abnormality has been identified as causative, and often multiple anatomic features are associated with OSA. Obesity increases the risk and severity of OSA and the severity of hypoxemia during sleep. Obesity and OSA are each independently associated with one another, with each contributing to the other’s severity. Fat distribution around the neck and airway has long been postulated to compromise the airway; however, obesity effects on metabolism, ventilation, and lung volume might be more important than volume and mass effects to the upper airway . Leptin and various inflammatory cytokines, which are either produced or influenced by adiposity, increase CO 2 response, and may contribute to central ventilatory sensitivity, and in turn result in further increased risk for obstructive breathing, as discussed below.
Craniofacial characteristics
Most studies assessing structure lack appropriate control groups, with groups differing not only in OSA but often also age, weight, ethnicity, and gender. “Normal” may be a subjective definition; however, few would argue that the skeletal and cartilage framework, which supports the soft tissues, which ultimately determine airway characteristics, are not critical. Framework abnormalities vary, and a constellation of abnormalities is consistently observed. Compared with nonsnoring controls, craniofacial variables associated with OSA include: increased distance of the hyoid bone from the mandibular plane, a decreased mandibular and maxillary projection, a downward and posterior rotation of the mandibular and maxillary growth, increased vertical length of the face, increased vertical length of the posterior airway, and increased cervical angulation. In the Wisconsin Cohort of Sleep Disordered Breathing study, two thirds or more of the variability of the apnea hypopnea index was explained by facial structure and obesity . In nonobese subjects, the major contributor was facial structure. Abnormalities in maxillary position and width explained much of OSA in all patient groups. From that study, it may be postulated that OSA is primarily a disorder of maxillary, not mandibular, development. An inferiorly based hyoid position has also been consistently associated with sleep disordered breathing. This displacement may signify abnormalities in airway length or increased tongue size.
Craniofacial characteristics
Most studies assessing structure lack appropriate control groups, with groups differing not only in OSA but often also age, weight, ethnicity, and gender. “Normal” may be a subjective definition; however, few would argue that the skeletal and cartilage framework, which supports the soft tissues, which ultimately determine airway characteristics, are not critical. Framework abnormalities vary, and a constellation of abnormalities is consistently observed. Compared with nonsnoring controls, craniofacial variables associated with OSA include: increased distance of the hyoid bone from the mandibular plane, a decreased mandibular and maxillary projection, a downward and posterior rotation of the mandibular and maxillary growth, increased vertical length of the face, increased vertical length of the posterior airway, and increased cervical angulation. In the Wisconsin Cohort of Sleep Disordered Breathing study, two thirds or more of the variability of the apnea hypopnea index was explained by facial structure and obesity . In nonobese subjects, the major contributor was facial structure. Abnormalities in maxillary position and width explained much of OSA in all patient groups. From that study, it may be postulated that OSA is primarily a disorder of maxillary, not mandibular, development. An inferiorly based hyoid position has also been consistently associated with sleep disordered breathing. This displacement may signify abnormalities in airway length or increased tongue size.
Nose
Nasal obstruction contributes to the presence and severity of OSA . Nasal blockage might
Reduce nasal afferent reflexes that help to maintain muscular tone of the upper airway
Augment the tendency for mouth opening, which destabilizes the lower pharyngeal airway (by posterior rotation, vertical opening, and inferior displacement of the hyoid)
Reduce humidification, increase mucus viscosity, and increase surface tension forces
Increase upstream airway resistance, predisposing to downstream airway collapse.
A multitude of pathologies cause nasal obstruction, and warrant appropriate evaluation.
Pharyngeal soft tissues
In adults, no single soft tissue structure contributes to OSA. The relative contribution of soft tissue size differs among individuals, and particularly among ethnic groups .
The size as well as position of the tongue is an important consideration in OSA. In the supine position, the tongue projects posteriorly and is counteracted by the tone of the genioglossal muscle. MRI volumetric studies have identified tongue size as a major predictor of OSA. Patients who have severe OSA, obesity, and marked lateral wall collapse demonstrated sagittally oriented airways that were speculated to result in unfavorable muscular mechanics for reopening the airway . Circular tubes are more efficient than those that are flat .
The soft palate is both longer and wider in OSA individuals. The tongue is larger, the oral to palatal airspace is smaller, and the posterior airspace behind both the tongue and palate is narrower. In some, the epiglottis is posteriorly placed.
Cross-sectional shape of the airway in apneics tends to be more elliptical rather than more circular. The elliptical shape increases the surface area of the airway and frictional resistance compared with a more circular conduit. This leads to a biomechanically weaker structure that is more easily collapsed at less negative pressures. Additionally, when the airway is oriented with its long axis in an anterior posterior direction (in the midsagittal plane), contraction of major airway dilators (such as the genioglossus muscle) is less effective.
Body/jaw position/gravity
Body position alters airway size and collapsibility. Airway size decreases following movements from sitting to supine, as well as lateral decubitus to supine . Changes are greater in OSA. Changes in tissue mass, lung volume, tracheal tug, and vascular volume from nonsupine to supine positioning may contribute to airway collapsibility, because they are independent (see discussion below). Gravity affects the lower pharynx and retro-epiglottic airway more than other segments during parabolic flight . Gravity has minimal effect on the position and airway of the upper pharynx and upper tongue base in nonapneic individuals. In apneic subjects, gravity likely contributes to obstruction at the lower pharynx. It may be speculated that because gravity alters the airway by affecting mass—as mass increases, gravity effects will increase.
Structural considerations
Balance of forces
A potentially large number of anatomic and physiologic processes must be integrated into a model of upper airway obstruction during sleep. One model that allows at least some integration is the concept of “balance of forces.” The balance of forces model allows an accurate description of how multiple variables alter upper airway size ( Fig. 3 ). Airway size is determined by both dilating and collapsing forces. Dilating forces include upper airway muscle tone, mechanical force of the airway wall structure, and positive intraluminal airway pressure. Collapsing forces include tissue mass, surface adhesive forces, and negative intraluminal pressures. The resulting difference in these forces is the distending force, which acts on the wall of the upper airway. When the distending force increases, the airway size increases; when it decreases, the airway size decreases.
The distending force of the upper airway is the transmural pressure (P tm ) of the airway. The equation P tm = P out − P in defines transmural pressure; P out represents the sum of the dilating the upper airway forces, and P in represents the sum of the collapsing forces. Another more clinically relevant means to conceive of the forces that act on the upper airway is by considering the skeletal airway structure as a constant and describing the dynamic forces as being either tissue pressures or luminal pressures (P tm = P tissue − P luminal ). Tissue pressure includes the forces from tissue mass, tissue elastance, surface tension, and neuromuscular dilating and collapsing forces. Luminal pressures include the segmental airway pressure (P airway ) and pressures relating to airflow (P flow ). Airway pressures may be dilating (ie, such as positive pressures during expiration or with the application of external positive pressure—continuous positive airway pressure [CPAP]) or collapsing (negative inspiratory pressure). Although seemingly esoteric, such a model (P tm = P luminal − P tissue ) provides a means of quantifying and describing upper airway collapse. Studies have been able to replicate a syndrome identical to OSAS in non-OSA subjects by applying negative intraluminal airway pressures to the upper airway during sleep. The airway pressure required to collapse the pharyngeal airway has been described by the critical closing pressure (P crit ) . When P tm = P crit , airway collapse occurs. Change in area/change in pressure (dA/dP) of the upper airway represents the tendency of the upper airway to collapse, and can be calculated allowing measurement of the intrinsic collapsibility of the upper airway. These measures, however, require controlling for both airflow velocity and muscular tone. Airflow velocity effects on luminal pressures are described by Bernoulli’s equation, and if velocity is zero, flow effects on the luminal pressure are eliminated (P luminal = P airway + 0). When neuromuscular tone is held constant, then tissue forces are constant (P tissue = k [constant] ). In this situation, measured airway pressure represents the distending or transmural pressure of the upper airway (P tm = P airway − k), and combined with measures of upper airway size, allows for calculation of airway compliance (dA/dP) independent of physiologic influences. Furthermore, airway pressure can be measured and manipulated (such as with nasal CPAP) to assess changes in airway size and compliance.
Starling resistor
In contrast to a structurally patent airway, where the pressure difference across the tube’s wall is of minimal importance and flow is determined by driving pressure, in sleep disordered breathing the tube wall characteristic becomes the major determinate of airway cross sectional area and air flow. The upper airway is a collapsible conduit with its flow described by the Starling resistor concept ( Fig. 4 ). The Starling Resistor concept builds upon Poiseuille’s law, which describes flow in noncollapsible tubes. Poiseuille’s law states: V = P 1 − P 2 /R (V = flow, P 1 = pressure upstream, P 2 = pressure downstream, P 1 − P 2 = driving pressure). The resistance component R is determined by length of the tube (L), fluid viscosity (η), and the radius R of the tube (R = resistance = 8ηL/πr 4 ). Changes in resistance relate inversely to changes in the area because viscosity and length are usually constant. For a rigid tube, flow is determined by the driving pressure across the tube (P 1 − P 2 ), Ohlm’s Law (V = I/R). This is in contrast to a collapsible tube, as is the case in the upper airway during sleep, where flow may be independent of driving pressure. Resistance changes, and is determined by airway size, which in turn fluctuates with airway luminal pressures, airflow, and all the other surrounding forces acting on the airway (such as described by the balance of forces). In the most basic form of collapsible tube “a simple collapsible tube” (defined as a tube having a wall without intrinsic structural forces), variations in airway size are determined primarily by the upstream and downstream airway pressures, flow, and the transmural pressures of the upper airway.
A simple collapsible tube is often modeled with a soft, thin-walled rubber drain placed between two rigid tubes. Three possible conditions of flow across this tube exist: unimpeded flow, flutter, and obstruction. These roughly correlate with the three basic clinical patterns of normal breathing, snoring, and obstruction.
In the human upper airway, the supraglottic pharynx is the collapsible tube with a transmural pressure (P tm ); the downstream pressure (P ds ) is negative inspiratory pressure (trachea), and upstream pressure (P us ) is ambient pressure (nose) (P crit ). During wakefulness, low negative inspiratory intraluminal pressures (ie, 5 cm H2O) combined with a large upper airway area (a positive transmural pressure) result in unimpeded flow. During sleep, although the balance of forces changes in “normals” without snoring or apnea, a structurally larger and stable upper airway remains patent. That is, the P crit remains lower than the P tm , preventing collapse. Because transmural pressure remains greater than both downstream and upstream pressures (P tm > P us > P ds ), the airway behaves more or less as a rigid tube; collapse does not occur and airway size and resistance are not altered.
In contrast, apneic subjects have a structurally smaller and more unstable upper airway, resulting in transmural pressures during sleep becoming lower than both downstream and upstream pressures (P us > P ds > P tm ). With transmural pressures more negative than the closing pressure of the airway during inspiration, airway size is zero. No flow occurs, regardless of the driving pressure across the tube.
An intermediate condition occurs in snorers. In snorers, upstream (ambient) pressures are higher than transmural pressures, but downstream pressures (P us > P tm > P ds ) are less. This results in flutter caused by a choke point of the airway exposed to alternating negative transmural pressure (ie, P ds ) and positive transmural pressure (ie, P us ). When the airway is open, it is exposed to downstream pressure, which acts to collapse the choke point. When the choke point is closed, it suddenly is no longer exposed to negative downstream pressures; instead, the segment is exposed to more positive upstream pressures. These pressures dilate and open the airway. An open airway is now exposed to negative downstream pressures and the airway collapses. Repeating of this cycle creates snoring at the choke point.
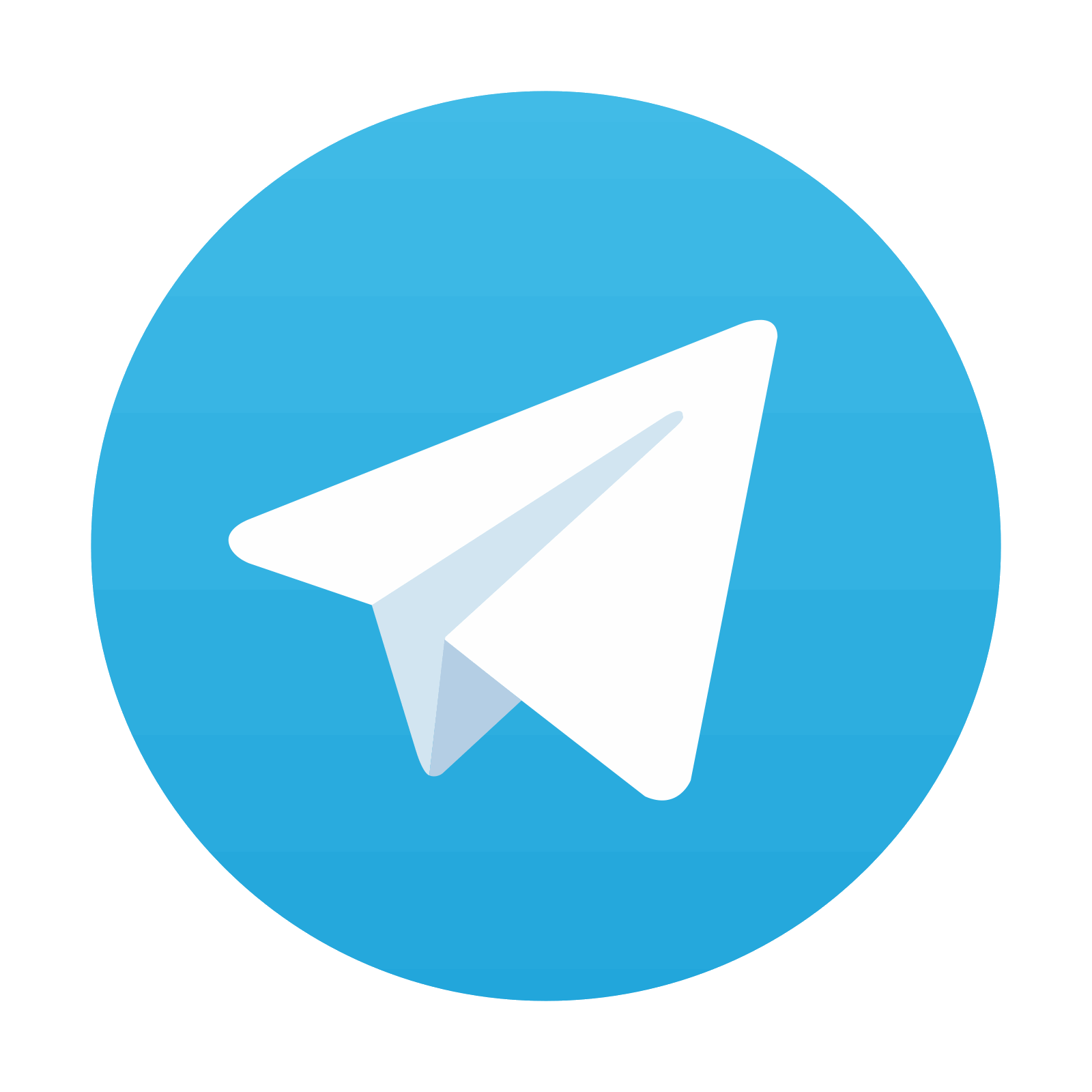
Stay updated, free articles. Join our Telegram channel
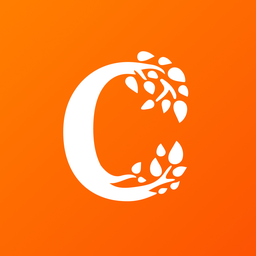
Full access? Get Clinical Tree
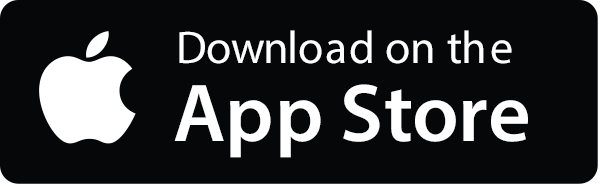
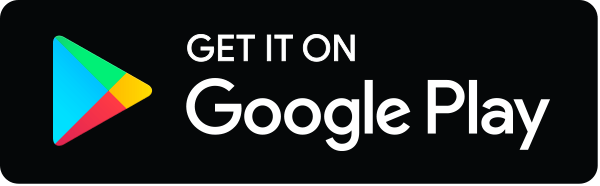