Fig. 1.1
Phases of human ROP. Drawing by James Gilman, CRA, FOPS. From ophthalmology, 2015 Jan;122(1):200–10, Hartnett ME, pathophysiology and mechanisms of severe retinopathy of prematurity
Term Versus Preterm Birth
To understand what goes wrong in retinopathy of prematurity (ROP), it is helpful to review some of the relevant and known events that occur in normal versus preterm birth, including the roles of oxygen and oxidative stress, and nutritional deficiencies, which have been linked to ROP.
Oxygen
In utero, it has been estimated that oxygen to the developing fetus is about 30–40 mm Hg, so the ambient environment after birth is relatively hyperoxic, particularly when supplemental oxygen is used for resuscitation [9]. High oxygen at birth has been shown to cause vasoattenuation and death to newly formed endothelial cells, [10] creating areas of retina devoid of capillary support. Human preterm infants in the US exposed to 100% oxygen prior to the technologic advances for monitoring and regulating oxygen, developed retrolental fibroplasia (RLF), now believed to be stage 5, the worst form of ROP [11]. The appearance of a white pupil represented a retrolental fibrovascular scar with an underlying retinal detachment. What actually occurred in the preterm infant retina prior to the development of RLF was limited because the Schepens/Pomerantzeff binocular indirect ophthalmoscope and other methods to observe the peripheral retina had not been widely adopted [12]. Therefore, experimental approaches using newborn animals exposed to conditions similar to what preterm infants then experienced were analyzed. From early experiments, it was hypothesized that high oxygen-induced capillary loss and avascular retina, and that removal from supplemental oxygen to ambient air, caused the avascular retina to become hypoxic and release angiogenic factors that led to aberrant angiogenesis and scarring [10]. These experiments also led to a phased approach description of ROP: phase 1, representing vasoobliteration, phase 2, vasoproliferation and phase 3, a fibrovascular phase in human and not animal models associated with retinal detachment [13]. When oxygen level was reduced, RLF virtually disappeared [11], but at too low an oxygen level there was increased risk of cerebral palsy and death [14]. Now, countries that lack resources for prenatal and perioperative care and oxygen regulation report ROP and hyperoxia-induced vasoattenuation, documented by fluorescein angiography, in large and older gestational aged infants than those in the US [15]. Advancements in neonatal care and oxygen regulation and monitoring have permitted infants of lower birth weights and younger gestational ages to survive. In countries implementing these changes, ROP is seen in infants of extremely low birth weight (<1000 g) and gestational ages (<28 wks), and the two-phase description of ROP has been refined to be delayed physiologic retinal vascular development with some vasoattenuation in phase 1 and vasoproliferation in phase 2.
Oxygen Stresses and Oxidation
The mechanisms whereby high oxygen affects newly developed capillaries and endothelial cells are not completely understood. Although the sensitivity of endothelial cells for oxygen lessens with development, hyperoxia-induced endothelial cell damage has still been reported in adult mice [16]. One potential mechanism whereby high oxygen damages endothelial cells is from mitochondrial-generated reactive oxygen species (ROS). In addition, other oxygen stresses, including fluctuations in oxygenation [17] and intermittent hypoxia [18], may lead to ROS generated from other sources, such as the activation of NADPH oxidase, a leading generator of ROS in endothelial cells. Also, reactive nitrogen species from activation of endothelial nitric oxide synthetase (eNOS) can generate nitric oxide (NO), which under certain circumstances forms damaging reactive nitrogen species (RNS), including peroxynitrite [19]. Nitric oxide can be beneficial to the preterm lungs and as an endothelial relaxing agent, and ROS are important in metabolism and for fighting invading microorganisms, but too many ROS or RNS can also cause direct tissue damage or indirect pathology from abnormal activation of signaling pathways. Generally, the reactive compounds are kept in check through antioxidant enzymes or nonenzymatic mechanisms, but the loss of balance between antioxidants and reactive species can lead to damage. There is evidence suggesting an imbalance occurs in preterm infants. Compared to infants born full-term, premature infants consume more glutathione (GSH), which provides a nonenzymatic oxidative reserve in red blood cells [20]. Hypoxia in complicated births is also believed to reduce the ability to quench mitochondrial ROS trapping [21]. In studies done in neonatal piglets, there is evidence that prostaglandins, such as E2, help preserve neural function following episodes of hypoxia, what occurs during progressive labor, but this mechanism may not be sufficient in abrupt preterm birth [22]. Also, preterm infants may be unable to express antioxidant enzymes until later age.
In regions that regulate oxygen, fluctuations in oxygenation have been measured in preterm infants in association with increased risk of ROP [17]. Fluctuations between 50 and 10% inspired oxygen in newborn rats cause similar arterial oxygen extremes in preterm infants and delayed physiologic retinal vascular development in experimental models, in part through the generation of ROS from activated NADPH oxidase [23–25] that causes endothelial cell death. Activation of NADPH oxidase in endothelial cells can also lead to vasoproliferation in a similar model of oxygen fluctuations [26].
Oxygen Trials
The practicality of regulating oxygen as a treatment for ROP has been tested in several multicenter clinical trials, including the Supplemental Therapeutic Oxygen to Prevent ROP (STOP-ROP) study [27], Surfactant Positive Airway Pressure Pulse Oximetry Randomized Trial (SUPPORT), Benefits of Oxygen Saturation Targeting Study II (BOOST II), and Canadian Oxygen Trial (COT) [28]. STOP-ROP failed to find a significant reduction in ROP from oxygen saturations between 96 and 99% when delivered for prethreshold ROP compared to 89–94%, except in a subgroup without evidence of plus disease [27]. Other clinical studies have reported either increased [29, 30] or decreased risk [31, 32] of ROP from high oxygen. The SUPPORT and BOOST II randomized clinical trials reported that oxygen saturation targets between 85 and 89% compared to 91–95% saturation were associated with increased mortality, but survivors of 85–89% oxygen saturation had a lower incidence of ROP. In the COT, which tested similar oxygen saturation targets in preterm infants of the same gestational ages, as did SUPPORT and BOOSTII, there was no difference in ROP or mortality. The COT was performed later in time from infants in different regions of the world compared to SUPPORT or BOOSTII, and also excluded from enrollment infants with pulmonary hypertension. From these studies, there does not appear to be consensus as to appropriate and safe oxygen saturation targets for preterm infants. The practicality of safely regulating oxygen in the individual preterm infant can be difficult because the phases of ROP are not as distinct as in experimental models, and oxygen affects other organs in the developing preterm infant [28], and ongoing analyses from these studies are underway.
Nutrition
Much of the nutritional support to the fetus occurs in the last trimester, such that when an infant is born prematurely, maternal support is lost [33]. Studies have shown the importance of insulin-like growth factor (IGF-1) and insulin-like growth factor binding protein 3 (IGF-1BP3) [34, 35] to infant development, which are reduced in the preterm infant in association with delayed physiologic retinal vascular development. In addition, omega-3 fatty acids, which make up the phospholipid rich tissue in neurons in the brain and photoreceptors in the retina, are reduced. Studies are ongoing to determine if recombinant human IGF-1 and IGF-1BP3 (rhIGF-I/rhIGFBP-3) will reduce ROP in infants. The phases in human ROP are not as distinct as in experimental models and there is concern that rhIGF-I/rhIGFBP-3 may be given to an infant who is about to develop vasoproliferative phase but who does not manifest signs of it. To reduce this, attempts are in place to increase the IGF-1/IGF-1BP3 only to levels normally found in infants of the gestational ages. Omega-3 FAs, if effective, can reduce both phase 1 and 2 in experimental models [36].
Phases of ROP and Phases of Experimental Oxygen-Induced Retinopathy
The initial two-phase hypothesis of human ROP described in the 1950s was based in large part on animal models in which retinal vascularization occurred after birth. Nonetheless, it is impossible to experiment on human preterm infant eyes safely and study mechanisms of human ROP; therefore, models of oxygen-induced retinopathy (OIR) are essential. It is important to consider the experimental question carefully when choosing a model or models (Fig. 1.2). The most representative model of human ROP today is the rat OIR model [37], which varies oxygen exposure between 50 and 10% to newborn rat pups and has been recently adapted to study molecular mechanisms using gene therapy techniques [6, 38–40]. Most studies have used the mouse OIR model, which exposes day 7 animals with inner plexus vascularization to high oxygen in order to damage already developed capillaries [41]. The mouse model is robust to study mechanisms of high oxygen-induced stress through the use of transgenic animals. The beagle OIR model uses high oxygen but is more similar to human ROP in that the retina continues to develop after birth. The beagle OIR model is also useful to translate pharmacologic interventions since the puppy eye size is closer to the human infant than is a rodent eye [42, 43].


Fig. 1.2
Phases of oxygen-induced Retinopathy (OIR). From ophthalmology, 2015 Jan;122(1):200–10, Hartnett ME, Pathophysiology and mechanisms of severe retinopathy of prematurity
Role of VEGF and Angiogenesis
Physiologic hypoxia is important in upregulating VEGF locally to promote normal retinal vascular development [4, 44, 45]. The parent VEGF mRNA has splice variants that have different biologic properties believed partly related to whether the translated proteins are cell associated, soluble, or have both characteristics [46]. VEGF splice variants are important in development and in aberrant angiogenesis. VEGF165 is both cell associated and can affect other retinal cells through soluble properties. VEGF164 (the analog of human VEGF165) is involved in vascular development in mice [47], but is upregulated by repeated fluctuations in oxygenation similar to the extremes in arterial oxygen in preterm infants who develop severe ROP [17]. In contrast, hypoxia alone increases the expression of soluble VEGF120 [25]. Inhibition of the rat VEGF164 [48] with a neutralizing antibody or by targeted gene therapy to introduce a shRNA to knock down VEGF164 only in Müller cells, reduced vasoproliferation but not physiologic retinal vascular development in the rat OIR model [6].
VEGF binds its receptors and triggers signaling of biochemical cascades to cause biologic events. There are three receptors and VEGFR2 is believed to be involved in much of the pathologic angiogenesis, although VEGFR1 is also important. Some studies support the idea that regulation of VEGFR2 signaling is important in ordering developmental angiogenesis. Over activation of VEGFR2 disordered the cleavage planes of dividing vascular cells causing them to take on a disordered pattern of growth [49]. Order was restored in experimental models with a neutralizing antibody to VEGF164 or through gene therapy to knock down Müller cell VEGF164. The evidence supports the notion that disordered endothelial cell growth enables cells to grow outside the plane of the retina and into the vitreous, whereas restoration of physiologic, ordered growth enables intraretinal blood vessel growth and reduces peripheral avascular retina. Over activation of VEGFR2 can occur by interactions with different receptors, such as the erythropoietin receptor (EPOR), or with other proteins including NOX4 [23], an isoform of NADPH oxidase, most likely through adaptor proteins. Downstream activation of the transcription factor, signal transducer, and activator of transcription 3 (STAT3) in endothelial cells causes vasoproliferation in experimental models [26].
Role of Anemia and Erythropoietin
Many preterm infants develop anemia of prematurity related to blood draws, inadequate erythropoiesis, and possible loss of blood at the time of delivery. Studies that tested the effect of restrictive versus liberal blood transfusions found no differences in the development of ROP [50, 51].
Erythropoietin (EPO) and its derivatives, such as darbepoietin, have been used for anemia of prematurity, but some clinical studies have associated severe ROP with the use of EPO [19, 20]. Vitreous EPO was increased in infants who underwent vitrectomies for stage 4 ROP compared to cataract surgery [21]. However, a recent clinical trial in infants given darbepoietin for neuroprotection found no effect compared to control on severe ROP [52], although, the number of infants with severe ROP was small. In addition, experimental studies found EPO administered early can stabilize newly formed retinal capillaries [23] and encourage physiologic retinal vascular development [24], but if given late can contribute to vasoproliferation [17, 22]. There is renewed interest in EPO for neuroprotection. EPO receptor can dimerize with itself or with the beta common receptor to form a tissue protective receptor [28]. However, experimental studies in the rat OIR model found very little beta common receptor expression in the retina in room air raised pups or those in the OIR model [40]. In contrast, repeated fluctuations in oxygenation activated not only the EPO receptor but also VEGFR2, which together formed a heterodimer and caused vitreous vasoproliferation in phase 2. Also, EPO was not increased in the OIR model but both EPOR expression and activation were increased. Furthermore, not only did EPO activate its receptor but VEGF also activated EPOR. With increasing expression of VEGF by oxygen stresses in ROP or through exogenous delivery of EPO, EPOR may be activated and interact with VEGFR2 to increase vasoproliferation. These observations may partly explain the discrepancies reported on EPO use in ROP. As with IGF-1 and other angiogenic factors that can reduce phase 1 ROP, the timing of delivery of the angiogenic agent, to avoid vasoproliferation, is complicated in the individual infant in whom the phases of ROP are not distinct.
Role of Oxidation, Inflammation, and Angiogenesis
Oxygen levels affect oxidation and the generation of ROS and RNS through mitochondria, NADPH oxidase, eNOS, and other enzymes. In addition, oxidative reserve is less in preterm than in full-term infants. High oxygen can increase the superoxide radical, and low oxygen may also increase superoxide [53] by slowing upstream events in the electron transport chain and increasing the concentration of oxygen donors. Repeated oxygen fluctuations can also activate enzymes that generate ROS, including NADPH oxidase. In addition, infection and inflammation can lead to signaling events that overlap with oxidative signaling [54], including angiogenesis and apoptosis [55, 56].
Inflammatory compounds are increased in low birth weight preterm infants in the antenatal period [57]. One family of metabolites includes the phospholipase A2 (PLA-2) enzymes that catalyze the hydrolysis of fatty acids from membrane phospholipids and are activated by oxidative stresses and hypoxia. PLA-2 is involved in experimental retinal neovascularization [58]. Activation of PLA-2 can then lead to the release of arachidonic acid, platelet activating factor, and lysophospholipids. Cyclo oxygenases (COX1 and COX2) can oxidize and catalyze arachidonic acid into the eicosanoids, including prostaglandins, prostacyclin, and thromboxanes that have been associated with both avascular retina and vasoproliferation in models of ROP and OIR [58]. Prostaglandin E2 activation of its receptor, EP4, caused Müller cell overexpression of VEGF and vasoproliferation in experimental OIR models [59].
Hypoxia, ROS, and inflammatory pathways through NFkB can stabilize hypoxia-inducible factor alpha 1 alpha (HIF1α). HIF1α then translocates to the nucleus initiating transcription of a number of angiogenic genes, including VEGF, erythropoietin, and angiopoietin 2, as examples [60]. HIF1α can also upregulate RTP801 to induce angiogenesis in a pathway independent of VEGF. RTP801 is strongly upregulated in ischemic cells of neuronal origin and can cause neuronal apoptosis [61]. Hypoxia also can increase the Krebs cycle metabolite, succinate, in retinal ganglion cells and trigger signaling through its receptor, GRPR91, to affect developmental angiogenesis and vasoproliferation in OIR models [7].
The cytochrome p450 monooxygenases (CYP) include a number of oxygen sensitive proteins that oxidize compounds, notably arachidonic acid. Autooxidation of arachidonic acid via CYP can produce lipid peroxides. CYP1B1 was also found to increase anti-angiogenic agent, thrombospondin 2 [62]. There is some evidence that CYP activation can exacerbate phospholipase A2 dependent injury [63] and may lead to a pathway associated with hyperoxia-induced endothelial apoptosis and vasoobliteration, seen in the mouse OIR model [64]. These events can contribute to avascular retina.
Generation of RNS, including peroxynitrite, can damage tissue but its precursor, NO, can be beneficial, and the difference may be related to the redox state of the tissue [65]. Other RNS, such as NO2 *, can lead to the isomerization of arachidonic acid to trans-arachidonic acid. Trans-arachidonic acid has been shown to contribute to hyperoxia-induced vasoobliteration through the upregulation of anti-angiogenic agent, thrombospondin-1 [56].
Although experimental approaches have shown that antioxidants can improve physiologic retinal vascular development, they have not reduced vasoproliferation. In human infants, antioxidants, n-acetyl cysteine [66], vitamin E [67] or lutein [68] also failed to safely reduce severe ROP. There can be different effects of ROS in different cells and evidence suggests that this is why broad inhibition of ROS may not always yield expected outcomes. In a rat OIR model in which rats were placed into 28% supplemental oxygen (supplemental oxygen OIR model) instead of room air, the antioxidant, apocynin, reduced vasoproliferation in part by inhibiting STAT3. In addition, vasoproliferation was nearly abolished with a systemic or intravitreal STAT3 inhibitor. However, in the rat OIR model in which rats were placed into room air (standard OIR model), broad STAT3 inhibition had no effect on vasoproliferation. The reason appeared to be based on the cell type in which STAT3 was activated. In contrast to the events in the supplemental oxygen OIR model, activation of STAT3 occurred in Müller cells by high VEGF in the standard OIR model and led to downregulation of EPO and delayed physiologic retinal vascular development. Exogenous EPO increased physiologic retinal vascular development by 40% but did not reduce vasoproliferation in phase 2, possibly because of the angiogenic effects of EPO. In the supplemental oxygen OIR model, VEGF was relatively downregulated compared to the standard OIR model reducing the effect of Müller cell STAT3 on EPO downregulation. In the supplemental oxygen OIR model, NADPH oxidase induced STAT3 was activated in endothelial cells and inhibition of STAT3 reduced vasoproliferation [26]. In the standard OIR model, STAT3 activation by VEGF mediated VEGFR2 activation in endothelial cells was in part through NADPH oxidase [26], as seen with high glucose [69]. This evidence supports the notion that reduction in vasoproliferation requires targeted endothelial cell STAT3 inhibition because broad inhibition of STAT3 may lead to upregulation of EPO that can act as an angiogenic factor. Studies are ongoing to look at ways to increase the effect of hypoxia inducible factors to support physiologic vascularization of the retina during high oxygen when hypoxia inducible factors are turned off during high oxygen treatment, which is often necessary for survival of the premature infant [70]. However, greater study is needed because many of these factors also affect processes important to the premature infant.
VEGF Signaling
Over activation of VEGF in the retina can disorder developmental angiogenesis and cause not only vasoproliferation into the vitreous, but also delayed physiologic retinal vascular development in phase 1. Inhibition of VEGF signaling can facilitate physiologic vascular development while inhibiting vasoproliferation. This finding was also seen in the clinical trial, Bevacizumab Eliminates the Angiogenic Threat of ROP (BEAT-ROP). However, in some infants treated with bevacizumab, persistent avascular retina and later vasoprolfieration occurred. Concerns exist that the dose of anti-VEGF agent, which was based on adult sized eyes, was too great for the preterm infant eye. In addition, the dilution of anti-VEGF agent that enters the blood volume in the preterm infant is much less than in the adult, and VEGF is important in neuroprotection, lung, and kidney development. More studies are needed to determine dose and safety.
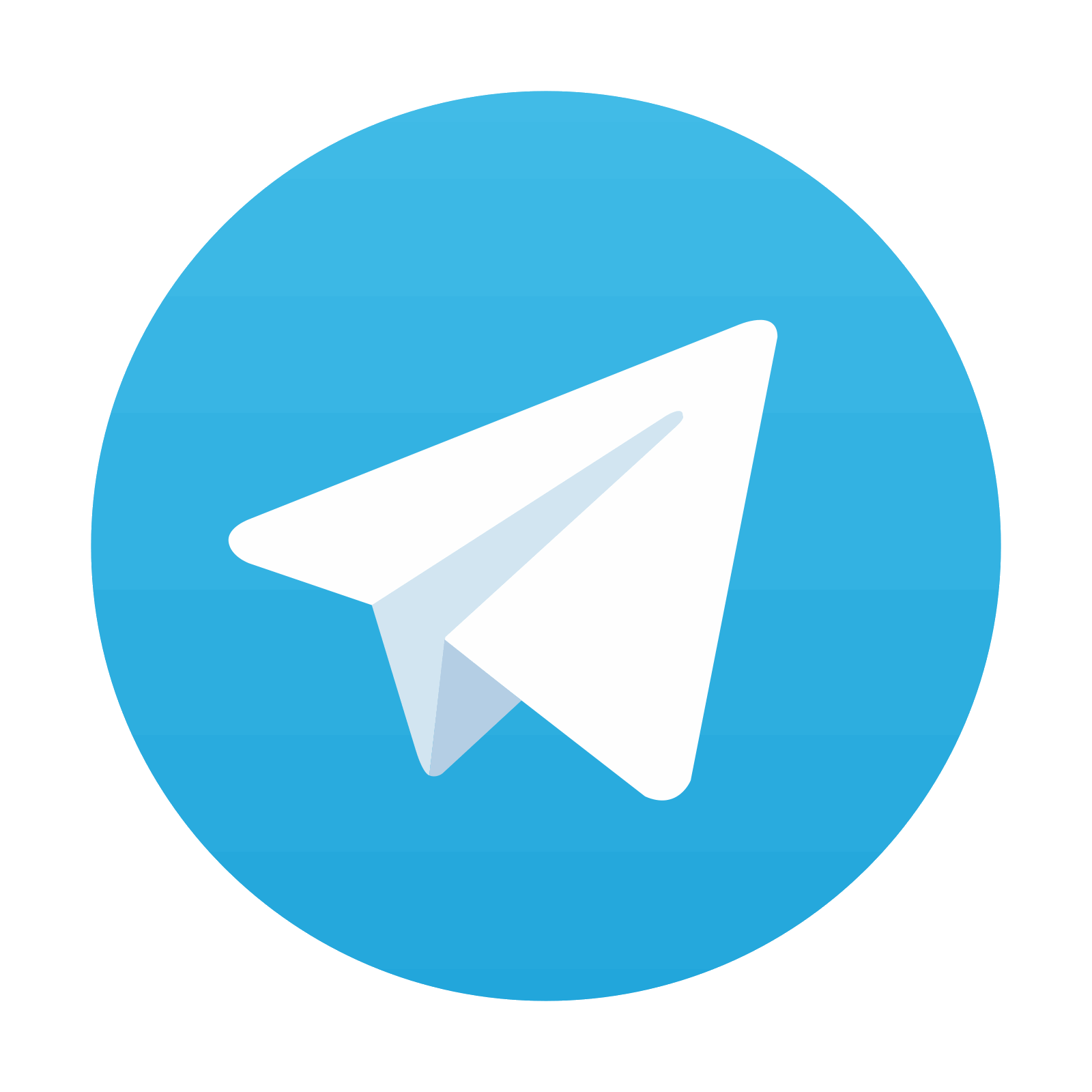
Stay updated, free articles. Join our Telegram channel
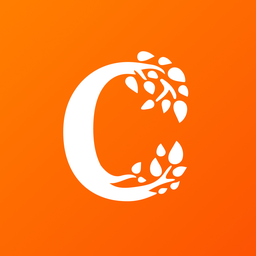
Full access? Get Clinical Tree
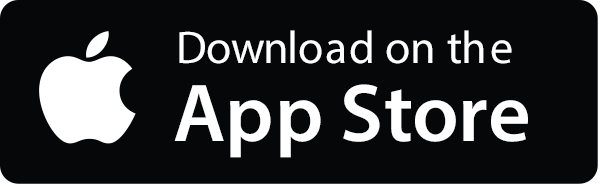
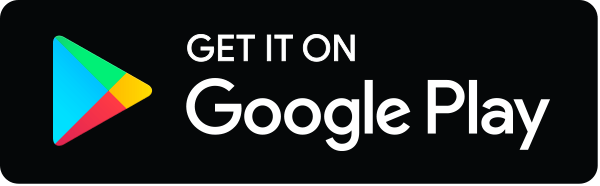