, Maneli Mozaffarieh1 and Hans Bebie2
(1)
Department of Ophthalmology, University of Basel, Basel, Switzerland
(2)
Institute for Theoretical Physics, University of Bern, Bern, Switzerland
Abstract
In the universe, oxygen is the third most abundant element after hydrogen and helium. Oxygen is synthesized at the end of the life of massive stars, when helium is fused into carbon, nitrogen, and oxygen nuclei. Stars burn out, explode, and expel the heavier elements into interstellar space. Later, oxygen plays a crucial role in the emergence of life. Oxygen is not always reactive to the same extent. The oxygen atom (O) is more reactive than the oxygen molecule (O2). To understand this, we will review some of the basics of oxygen. The oxygen atom is depicted in Fig. 9.1.
9.1 The Oxygen Atom
In the universe, oxygen is the third most abundant element after hydrogen and helium. Oxygen is synthesized at the end of the life of massive stars, when helium is fused into carbon, nitrogen, and oxygen nuclei. Stars burn out, explode, and expel the heavier elements into interstellar space. Later, oxygen plays a crucial role in the emergence of life. Oxygen is not always reactive to the same extent. The oxygen atom (O) is more reactive than the oxygen molecule (O2). To understand this, we will review some of the basics of oxygen. The oxygen atom is depicted in Fig. 9.1.


Fig. 9.1
Oxygen atom, which has eight protons, eight neutrons, and eight electrons
The eight electrons of the oxygen atom fill the “s” and “p” orbitals. The names “s” and “p” indicate the orbital shape and are used to describe the electron configurations. S orbitals are spherically symmetric around the nucleus, whereas p orbitals are rather like two identical balloons tied at the nucleus. The electron configuration for the oxygen atom reads as follows: 1s2 2s2 2p4. There are two electrons in the first shell and six in the second (Fig. 9.2). In the second shell, two electrons occupy an s-type orbital and four occupy p-type orbitals. Given that a p-type orbital has a capacity of six electrons, the oxygen atom falls short by two electrons of its “wanting” to fill its outermost shell to its full natural capacity.


Fig. 9.2
Electron configuration of the oxygen atom. Two electrons occupy the first shell of the oxygen atom and six electrons occupy the second shell (electron configuration: 1s2 2s2 2p4)
This explains the high reactivity of the oxygen atom. Oxygen is, after fluorine, the most electronegative element. The electronegativity of an element describes its “electron hunger.” Atoms or molecules with an unpaired electron in their outer shell are called free radicals. The oxygen atom is a free radical. Since the two 2p orbitals (each containing a lone electron) are not full, the oxygen atom tries to become stable by reacting with other atoms and trying to add the electron of the other atom to its own shell. This makes the oxygen atom highly reactive. In nature, an oxygen atom typically steals away an electron from one or two other atoms to form a molecule, such as water (H2O). To form an oxygen molecule, each oxygen atom donates two electrons to the other oxygen atom. In the case of the formation of water, each hydrogen atom “donates” one electron to the oxygen (Fig. 9.3).


Fig. 9.3
Example of a redox reaction. When hydrogen and oxygen bind, the electron of the hydrogen is “donated” to the oxygen. Hydrogen is, therefore, oxidized, whereas oxygen is reduced
This is an example of a redox reaction where hydrogen atom is oxidized (“loses electrons”) and oxygen is reduced (“gains electrons”). This electron transfer sets energy free; in other words, it releases heat, which is why this reaction is called exothermic. We can, therefore, also say that water is formed when hydrogen is “burned” by oxygen.
Similar to the oxygen atom, molecular oxygen also has two unpaired electrons in its last orbital that have the same spin (Fig. 9.4). Interestingly, however, the oxygen molecule, although a bi-radical, is only minimally reactive because the unpaired electrons in the oxygen molecule have the same spin. Thus, for the oxygen molecule to be able to react, it would need another molecule or atom with two unpaired electrons with a parallel spin opposite to that of the oxygen molecule. The latter will only rarely occur. Hence, the oxygen molecule is minimally reactive (spin restriction).


Fig. 9.4
Molecular oxygen, which also has two unpaired electrons in its last orbital that have the same spin direction. To bind with another molecule, it would need another molecule or atom with two unpaired electrons with a parallel spin opposite to that of the oxygen molecule. This will rarely occur, explaining why molecular oxygen is minimally reactive
As oxygen is almost always needed in biological energy metabolism, we shall discuss the role of oxygen in more detail.
9.2 Oxygen and Energy Production
Oxygen is one of the most important molecules required for human life. One of the key ways for a cell to gain useful energy is by aerobic respiration (in other words, by oxidizing high-energy molecules). In environments, however, where oxygen is not sufficiently available, both primitive organisms and cells in our body can metabolize glucose by fermentation without using oxygen. This process also yields energy, though in lower amounts, and produces byproducts such as lactic acid.
9.3 Biochemical Reactions of Oxygen
Combustible material (e.g., sugar or wood) can be found anywhere on earth. Fortunately, most atmospheric oxygen (molecular oxygen) is inert, meaning that it reacts very sluggishly with other molecules. The oxygen in the air we breathe is normally in its “ground” (not energetically excited) state. However, for use in mitochondria (see Sect. 14.3), it does not need to be activated since mitochondria have special machinery that donates electrons to the oxygen molecule. If, however, oxygen reacts outside the oxidative phosphorylation pathway, it needs to be activated. This activation of oxygen may occur by two different mechanisms: either through the absorption of sufficient energy to reverse the spin on one of the unpaired electrons or through monovalent reduction (Fig. 9.5).


Fig. 9.5
Activation of ground-state oxygen, which can be either activated physically (direct excitation by light) to form the singlet oxygen or it can be activated chemically by a reduction reaction (one electron reduction of dioxygen) to form the superoxide anion. (From Mozaffarieh M, Flammer J (2009) Ocular blood flow and glaucomatous optic neuropathy. Springer Publ, Berlin. With permission)
Thus, if ground-state oxygen absorbs sufficient energy to reverse the spin of one of its unpaired electrons, the two unpaired electrons now have opposite spins. In this more reactive form of oxygen, namely singlet oxygen (1O2), one of these unpaired electrons has a changed spin direction (Fig. 9.6).


Fig. 9.6
Singlet oxygen. If ground-state oxygen absorbs sufficient energy to reverse the spin of one of its unpaired electrons, the two unpaired electrons now have opposite spins. This more reactive form of oxygen is called singlet oxygen (1O2)
Singlet oxygen, being much more reactive than ground-state oxygen, reacts destructively with molecules with double bonds.
The second mechanism of activation is by the stepwise monovalent reduction of oxygen that gives rise to superoxide anion radical (O2•−), hydrogen peroxide (H2O2), and, finally, water (H2O). The situation is similar to that of a burning fire. To make a fire, we first need to transfer heat to a combustible material because the fire contains a cloud of electrons that facilitates the transfer of electrons to oxygen.
During combustion (burning), electrons are transferred from, for example, hydrogen or carbon to oxygen, resulting in the final product of water or carbon dioxide, respectively. We inhale oxygen and exhale carbon dioxide (the carbon comes from sugar or fat). To close the circuit and reach the “steady state” of these molecules in the atmosphere, oxygen must be regenerated by plants with the help of photosynthesis.
The term “photosynthesis” simply implies “synthesis” with the necessary energy coming from light (“photo”). The energy from sunlight is absorbed by green plants with the aid of the green pigment (chlorophyll) (Fig. 9.7).


Fig. 9.7
Structure of chloroplast. Green plants are green because they contain the pigment chlorophyll, found in the thylakoid sacs of the chloroplast. Left: Cross-section of a leaf. Middle: Thylakoids within the stroma of the chloroplast. Right: Thylakoids are arranged in stacks called grana
Light absorbed by the chlorophyll is in the visible spectrum, which is a small part of the electromagnetic spectrum, as shown in Fig. 9.8.


Fig. 9.8
Visible light. From among the broad spectrum of electromagnetic waves, only a small portion is perceived as light
The longer the wavelength of visible light is the more red the color will be. Likewise, the shorter wavelengths are toward the violet side of the spectrum. Since not all wavelengths of visible light are absorbed by chlorophyll, the leaves of plants appear green rather than black. Chlorophyll plant pigments are capable of absorbing light. Their light absorption spectrum is shown in the illustration in Fig. 9.9.


Fig. 9.9
Chlorophyll light absorption. Chlorophyll pigments absorb light in the blue (short-wavelength) and the red (long-wavelength) regions of the visible light spectrum, as marked by A. The wavelengths of light that are not absorbed include the remaining green-yellow colors, between 500 and 600 nm (marked by R), which explains why plants appear green. The wavelengths of light that are not absorbed include the remaining green-yellow colors, between 500 and 600 nm
To regenerate oxygen, the chlorophyll molecules in plants need to take away an electron from the oxygen atom bound within a molecule, which in the case of photosynthesis is water. Prior to this, however, the chlorophyll molecule itself needs to lose an electron, as only the oxidized chlorophyll can take an electron away from oxygen. Chlorophyll is oxidized with the help of sunlight energy. However, one photon of light does not give the chlorophyll molecule enough energy to lose an electron. For this reason, the energy of several photons is summed up in the so-called “antenna” of chlorophyll molecules (Fig. 9.10).

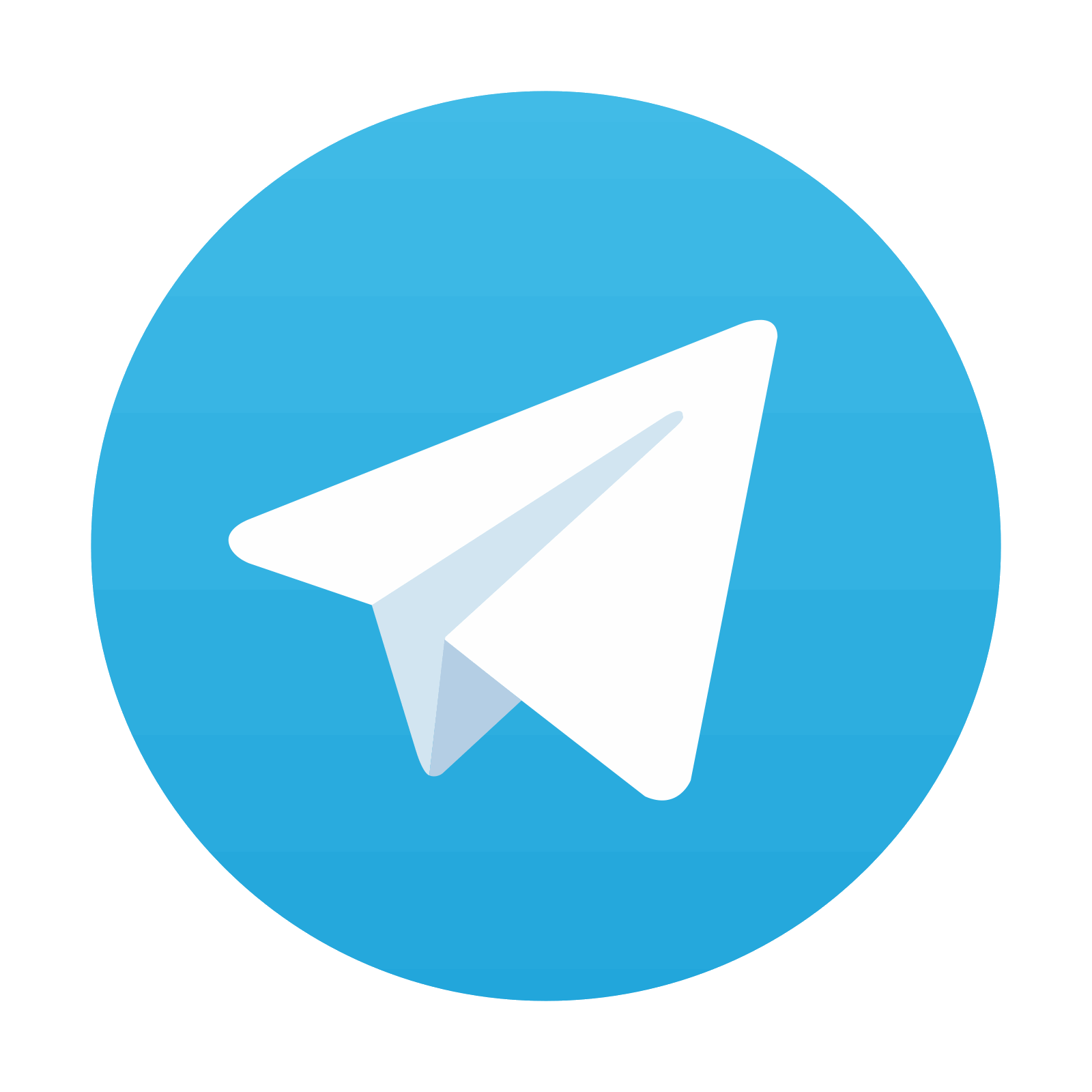

Fig. 9.10
Accumulation of energy by chlorophyll. The different chlorophyll molecules are arranged to form a light-harvesting complex also known as the “antenna” of chlorophyll molecules
< div class='tao-gold-member'>
Only gold members can continue reading. Log In or Register a > to continue
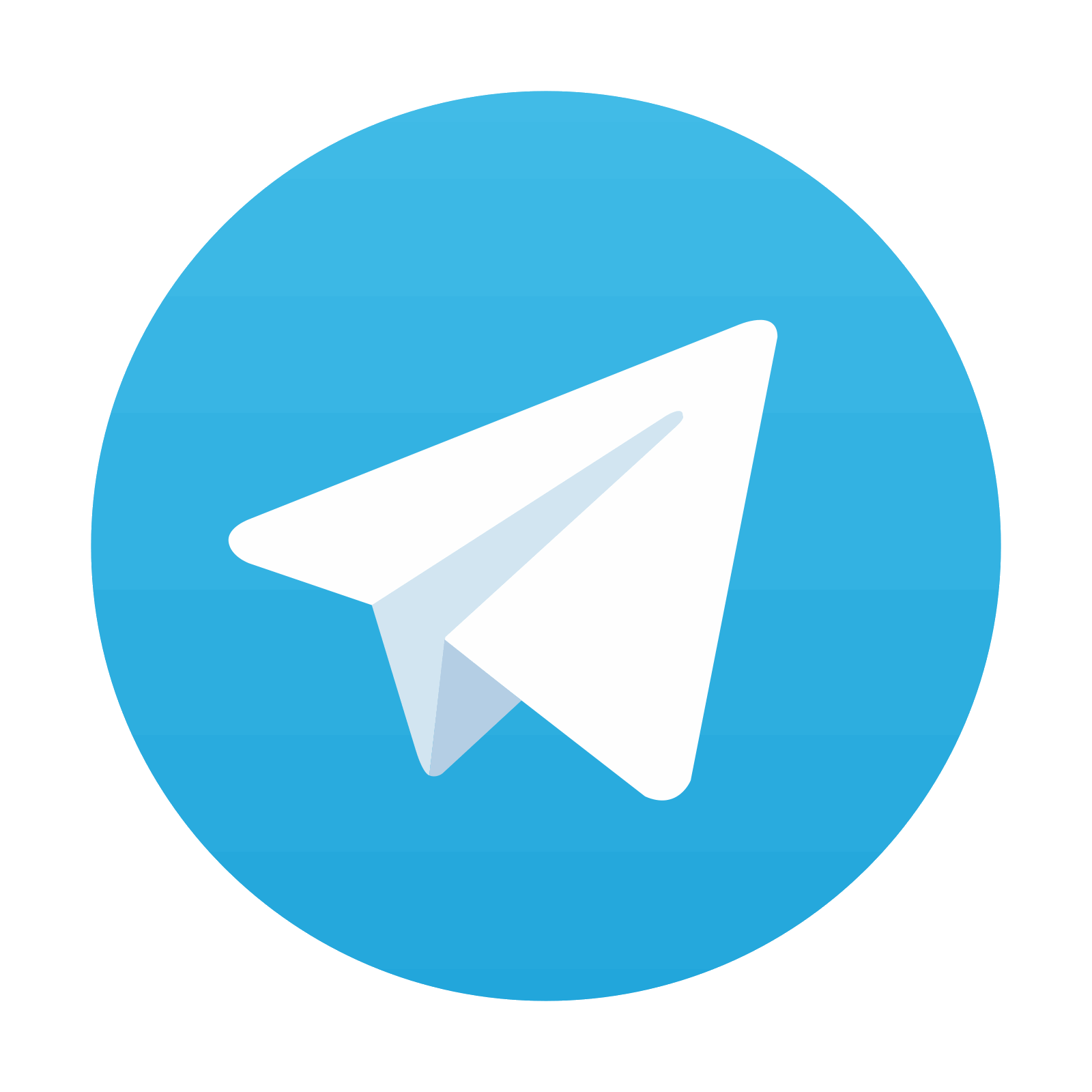
Stay updated, free articles. Join our Telegram channel
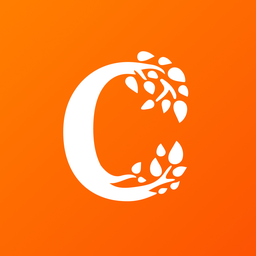
Full access? Get Clinical Tree
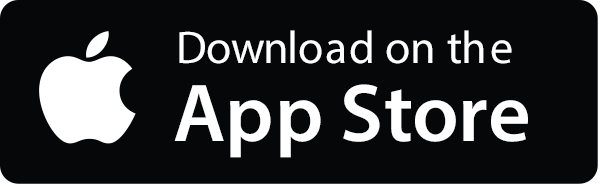
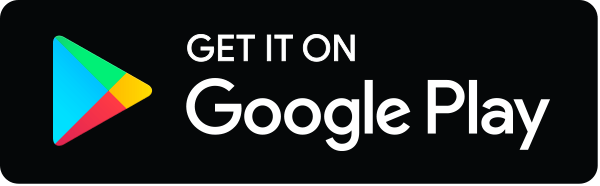
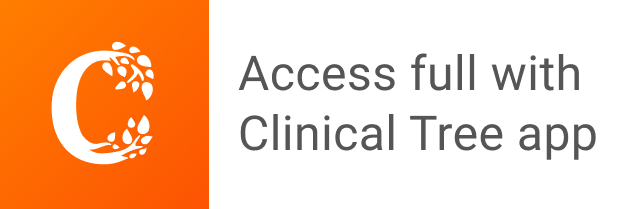